60 Neurophysiology
Principles of Electricity
When a neuron is stimulated, an electrical impulse is generated and conducted along the length of its axon. This process, called action potential, underlies many nervous system functions.
Learning Objectives
Identify principles of electricity that are important in neurophysiology
Key Takeaways
Key Points
- Voltage and current are two important factors to consider in the study of neurons.
- The flow of electrical charge from one point to another is called current.
- Across the cellular membranes, potential difference is established between the outside of a cell and the inside of the cell which can affect the flow of current across the cell membrane.
Key Terms
- Voltage: The measure of potential energy generated by separated charge.
- current: The flow of electrical charge from one point to another.
- resistance: Hindrance to the flow of charge.
Voltage and current are two important factors to consider in the study of neurons. Voltage is the measure of potential energy generated by separated charge. It is measured in volts or millivolts. The greater the difference in charge between two points, the higher the voltage. Voltage is equal to the work that would have to be done per unit charge to move the charge between two points against a static electric field. Voltage may represent either a source of energy (electromotive force) or lost or stored energy (potential drop).
The flow of electrical charge from one point to another is called current. The amount of charge that moves between two points depends on two factors: voltage and resistance. Resistance is the hindrance to the flow of charge. Some substances with high resistance are insulators, like the myelin sheath.
In the body, electrical currents reflect the flow of ions across cell membranes. Since there is a slight difference in the number of positive and negative ions on the two sides of the cellular plasma membrane, there is a potential difference across the membranes. Diffusion arises from the statistical tendency of particles to redistribute from regions where they are highly concentrated to regions where the concentration is low. The difference in potential established between the outside of a cell and the inside of the cell can affect the flow of current across the cell membrane.
Cellular Membrane: Differences in concentration of ions on opposite sides of a cellular membrane lead to a voltage called the membrane potential. Many ions have a concentration gradient across the membrane, including potassium (K+), which is at a high inside and a low concentration outside the membrane. Sodium (Na+) and chloride (Cl–) ions are at high concentrations in the extracellular region and low concentrations in the intracellular regions. These concentration gradients provide the potential energy to drive the formation of the membrane potential.
In electrically active tissue, the potential difference between any two points can be measured by inserting an electrode at each point and connecting both electrodes to to a specialized voltmeter.
Ion Channels
Ion channels are membrane proteins that allow ions to travel into or out of a cell.
Learning Objectives
Distinguish between types of ion channels
Key Takeaways
Key Points
- Ion channels can be voltage-sensitive, ligand-gated, or mechanically-gated in nature.
- Ligand-gated ion channels open when a chemical ligand such as a neurotransmitter binds to the protein.
- Voltage channels open and close in response to changes in membrane potential.
- Mechanically-gated channels open in response to physical deformation of the receptor, as in sensory receptors of touch and pressure.
Key Terms
- leakage channel: The simplest type of ion channel, with more or less constant permeability.
- ligand-gated channel: A group of transmembrane ion channels that open or close in response to the binding of a chemical messenger (ligand) such as a neurotransmitter.
- ion pump: Critical membrane proteins that carry out active transport by using cellular energy (ATP) to “pump” the ions against their concentration gradient.
Examples
Voltage-gated sodium ion channels contribute to the “spike” of a neuron’s action potential.
Properties of Ion Channels
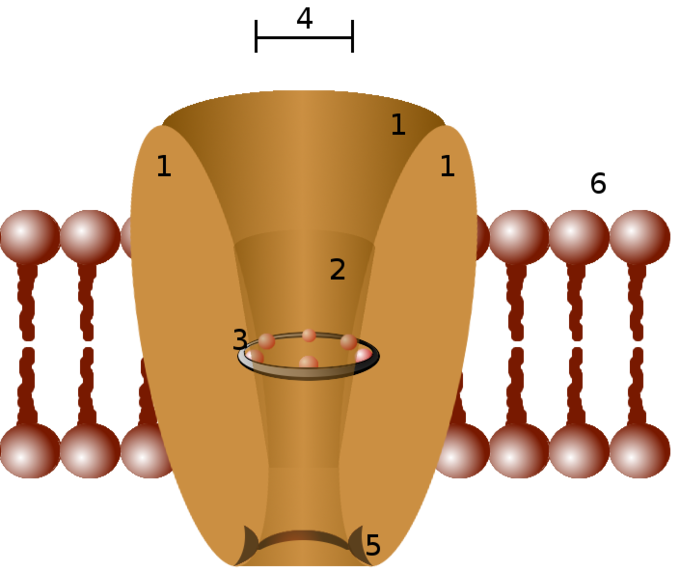
Plasma membrane is studded with a variety of membrane proteins that act as ion channels. Each channel only allows certain types of ions to pass across the membrane. Most channels are specific (selective) for one ion. The channel pore is typically so small that ions must pass through it in single file.
A channel may have several different states (corresponding to different conformations of the protein), but each such state is either open or closed. In general, closed states correspond either to a contraction of the pore—making it impassable to the ion—or to a separate part of the protein, stoppering the pore. When a channel is open, ions permeate through the channel pore down the transmembrane concentration gradient for that particular ion. Rate of ionic flow through the channel is determined by the maximum channel conductance and electrochemical driving force for that ion.
Types of Ion Channels
Ion channels can be classified by how they respond to their environment. For example, ion channels can be voltage-sensitive in that they open and close in response to the voltage across the membrane. Ligand-gated channels form another important class; these ion channels open and close in response to the binding of a ligand molecule such as a neurotransmitter. Other ion channels open and close with mechanical forces. Still others, such as those of sensory neurons, open and close in response to other stimuli, such as light, temperature, or pressure. The most common types of ion channels are described below.
Leakage Channels
Leakage channels are the simplest type of ion channel, in that their permeability is more or less constant. The types of leakage channels with the greatest significance in neurons are potassium and chloride channels. Although they are the simplest in theory, most conduct better in one direction than the other (they are rectifiers) and some are capable of being shut off by ligands even though they do not require ligands in order to operate.
Gated Channels
There are three main types of gated channels: chemically-gated or ligand-gated channels, voltage-gated channels, and mechanically-gated channels.
Ligand-gated ion channels are channels whose permeability is greatly increased when some type of chemical ligand binds to the protein structure. A large subset function as neurotransmitter receptors—they occur at postsynaptic sites, and the chemical ligand that gates them is released by the presynaptic axon terminal. Ligand-gated channels can be activated by ligands that appear in the extracellular area or by interactions on the intracellular side.
A Ligand-gated Ion Channel: Ligand-gated ion channels (LGICs) are one type of ionotropic receptor or channel-linked receptor. They are a group of transmembrane ion channels that open or close in response to the binding of a chemical messenger (ligand), such as a neurotransmitter.
Voltage-gated ion channels, also known as voltage-dependent ion channels, are channels whose permeability is influenced by the membrane potential. They form another very large group, with each member having a particular ion selectivity and a particular voltage dependence. Many are also time-dependent—in other words, they do not respond immediately to a voltage change, but only after a delay. Voltage-gated channels are essential for the generation and propagation of action potentials.
Ion pumps are not ion channels, but are critical membrane proteins that carry out active transport by using cellular energy (ATP) to “pump” the ions against their concentration gradient. Such ion pumps take in ions from one side of the membrane (decreasing its concentration there) and release them on the other side (increasing its concentration there).
Ion pump example: Example of primary active transport, where energy from hydrolysis of ATP is directly coupled to the movement of a specific substance across a membrane independent of any other species.
Resting Membrane Potentials
The potential difference in a resting neuron is called the resting membrane potential.
Learning Objectives
Describe the resting membrane potential of cells
Key Takeaways
Key Points
- The potential difference in a resting neuron is called the resting membrane potential.
- The value of the resting membrane potential varies from -40mV to -90mV in a different types of neurons.
- Most of the time, the difference in ionic composition of the intracellular and extracellular fluids and difference in ion permeability generates the resting membrane potential difference.
- The Goldman formula essentially expresses the membrane potential as an average of the reversal potentials for the individual ion types weighted by permeability
Key Terms
- resting membrane potential: The potential difference in a resting neuron that causes its membrane to be polarized.
- Goldman equation: Models the interactions that generate resting membrane potential.
The potential difference in a resting neuron is called the resting membrane potential. This causes the membrane to be polarized. The value of the resting membrane potential varies from −40mV to −90mV in a different types of neurons.
The resting membrane potential exists only across the membrane. Most of the time, the difference in ionic composition of the intracellular and extracellular fluids and difference in ion permeability generates the resting membrane potential difference.
The interactions that generate the resting potential are modeled by the Goldman equation. It is based on the charges of the ions in question, as well as the difference between their inside and outside concentrations and the relative permeability of the plasma membrane to each ion where:
[latex]\displaystyle{E}_{m}=\frac{RT}{F}\text{ln}\left(\frac{P_{\text{K}}\left[\text{K}^{+}\right]_{\text{out}}+P_{\text{Na}}\left[\text{NA}^{+}\right]_{\text{out}}+P_{\text{Cl}}\left[\text{Cl}^{-}\right]_{\text{in}}}{P_{\text{K}}\left[\text{K}^{+}\right]_{\text{in}}+P_{\text{Na}}\left[\text{Na}^{+}\right]_{\text{in}}+P_{\text{Cl}}\left[\text{Cl}^{-}\right]_{\text{out}}}\right)[/latex]
Goldman equation: R is the universal gas constant, equal to 8.314 joules·K−1·mol−1 T is the absolute temperature, measured in kelvins (= K = degrees Celsius + 273.15) F is the Faraday constant, equal to 96,485 coulombs·mol−1 or J·V−1·mol−1
The three ions that appear in this equation are potassium (K+), sodium (Na+), and chloride (Cl−). The Goldman formula essentially expresses the membrane potential as an average of the reversal potentials for the individual ion types, weighted by permeability. In most animal cells, the permeability to potassium is much higher in the resting state than the permeability to sodium. Consequently, the resting potential is usually close to the potassium reversal potential.
Membrane Potentials as Signals
The membrane potential allows a cell to function as a battery, providing electrical power to activities within the cell and between cells.
Learning Objectives
Describe how membrane potentials are used as signals
Key Takeaways
Key Points
- A membrane potential is the difference in electrical potential between the interior and the exterior of a biological cell.
- In electrically excitable cells, changes in membrane potential are used for transmitting signals within the cell.
- The opening and closing of ion channels can induce changes from the resting potential.
- Depolarization is when the interior voltage becomes more positive and hyperpolarization is when it becomes more negative.
Key Terms
- action potential: A short-term change in the electrical potential that travels along a cell such as a nerve or muscle fiber.
- depolarization: A change in a cell’s membrane potential, making it more positive or less negative, that may result in generation of an action potential.
- graded potentials: These vary in size and arise from the summation of the individual actions of ligand-gated ion channel proteins, and decrease over time and space.
Examples
In neurons, a sufficiently large depolarization can evoke an action potential in which the membrane potential changes rapidly.
Membrane Potentials
Membrane potential (also transmembrane potential or membrane voltage) is the difference in electrical potential between the interior and the exterior of a biological cell. All animal cells are surrounded by a plasma membrane composed of a lipid bilayer embedded with various protein types. The membrane serves as both an insulator and a semi-permeable diffusion barrier to the movement of ions. Ions are moved across the cell membrane either through active (using energy) or passive (not using energy) transport.
For example, ion transporter/pump proteins actively push ions across the membrane to establish concentration gradients across the membrane and ion channels allow ions to move across the membrane down those concentration gradients, a process known as facilitated diffusion.
Virtually all eukaryotic cells (including cells from animals, plants, and fungi) maintain a nonzero transmembrane potential, usually with a negative voltage in the cell interior compared to the cell exterior.
The Membrane Potential as a Signal
The membrane potential has two basic functions. First, it allows a cell to function as a battery, providing power to operate a variety of “molecular devices” embedded in the membrane. Second, in electrically excitable cells such as neurons and muscle cells, it is used for transmitting signals between different parts of a cell. Signals are generated by opening or closing of ion channels at one point in the membrane, producing a local change in the membrane potential that causes electric current to flow rapidly to other points in the membrane.
In non-excitable cells, and in excitable cells in their baseline states, the membrane potential is held at a relatively stable value called the resting potential. For neurons, typical values of the resting potential range from –70 to –80 millivolts; that is, the interior of a cell has a negative baseline voltage of a bit less than one tenth of a volt. The opening and closing of ion channels can induce a departure from the resting potential. This is called a depolarization if the interior voltage becomes more positive (say from –70 mV to –60 mV), or a hyperpolarization if the interior voltage becomes more negative (say from –70 mV to –80 mV). The changes in membrane potential can be small or larger (graded potentials) depending on how many ion channels are activated and what type they are. In excitable cells, a sufficiently large depolarization can evoke an action potential in which the membrane potential changes rapidly and significantly for a short time (on the order of 1 to 100 milliseconds), often reversing its polarity. Action potentials are generated by the activation of certain voltage-gated ion channels.
Action potential: A. Schematic and B. actual action potential recordings. The action potential is a clear example of how changes in membrane potential can act as a signal.
The Action Potential and Propagation
Neurons typically send signals over long distances by generating and propagating action potentials over excitable axonal membrane.
Learning Objectives
Describe the stages of an action potential and its propogation
Key Takeaways
Key Points
- Action potential is a brief reversal of membrane potential in which the membrane potential changes from -70mV to +30mV
- The action potential has three main stages: depolarization, repolarization, and hyperpolarization.
- Depolarization is caused when positively charged sodium ions rush into a neuron with the opening of voltage-gated sodium channels.
- Repolarization is caused by the closing of sodium ion channels and the opening of potassium ion channels.
- Hyperpolarization occurs due to an excess of open potassium channels and potassium efflux from the cell.
Key Terms
- action potential.: A brief reversal of membrane potential.
- repolarization: Also called the falling phase,
caused by the slow closing of sodium channels and the opening of voltage-gated potassium channels. - absolute refractory period: The period from the opening of the sodium channels until the sodium channels begin to reset.
- hyperpolarization: A phase where some potassium channels remain open and sodium channels reset.
- depolarization: Also called the rising phase, when positively charged sodium ions (Na+) suddenly rush through open voltage-gated sodium channels into a neuron.
Action Potential
Action potential is a brief reversal of membrane potential where the membrane potential changes from -70mV to +30mV. When the membrane potential of the axon hillock of a neuron reaches threshold, a rapid change in membrane potential occurs in the form of an action potential.
This moving change in membrane potential has three phases. First is depolarization, followed by repolarization and a short period of hyperpolarization. These three events happen over just a few milliseconds.
Action potential: A. Schematic and B. actual action potential recordings. The action potential is a clear example of how changes in membrane potential can act as a signal.
- The depolarization, also called the rising phase, is caused when positively charged sodium ions (Na+) suddenly rush through open voltage-gated sodium channels into a neuron. As additional sodium rushes in, the membrane potential actually reverses its polarity. During this change of polarity the membrane actually develops a positive value for a moment (+40 millivolts).
- The repolarization or falling phase is caused by the slow closing of sodium channels and the opening of voltage-gated potassium channels. As a result, the membrane permeability to sodium declines to resting levels. As the sodium ion entry declines, the slow voltage-gated potassium channels open and potassium ions rush out of the cell. This expulsion acts to restore the localized negative membrane potential of the cell.
- Hyperpolarization is a phase where some potassium channels remain open and sodium channels reset. A period of increased potassium permeability results in excessive potassium efflux before the potassium channels close. This results in hyperpolarization as seen in a slight dip following the spike.
The propagation of action potential is independent of stimulus strength but dependent on refractory periods. The period from the opening of the sodium channels until the sodium channels begin to reset is called the absolute refractory period. During this period, the neuron cannot respond to another stimulus, no matter how strong.
The Synapse
A synapse is a structural junction that mediates information transfer from one neuron to the next or from one neuron to an effector cell as in muscle or gland.
Learning Objectives
Describe the role of a synapse in nervous tissue
Key Takeaways
Key Points
- In the nervous system, a synapse is a structure that permits a neuron to pass an electrical or chemical signal to another cell.
- The neuron conducting impulses towards the synapse is called pre-synaptic neuron. The neuron transmitting the electrical impulse away from the synapse is called post-synaptic neuron, if the post-synaptic cell is not neuronal it is sometimes referred to as an effector cell.
- There are two varieties of synapses: electrical and chemical synapse based on the type of transmission.
- Chemical synapses rely on the secretion of neurotransmitters across a synaptic cleft between cells. Receptors on the post-synaptic cell bind these neurotransmitters and induce a signalling response.
- Electrical synapses form when the plasma membranes of two neurons are fused, and punctured by gap junction proteins, allowing for the diffusion of ions across the plasma cell membrane.
- Chemical synapses are slower than electrical synapses, but allow for gain, or amplification of signal.
Key Terms
- axon: A long, slender projection of a nerve cell, or neuron, that typically conducts electrical impulses away from the neuron’s cell body.
- synapse: A structure that permits a neuron to pass an electrical or chemical signal to another cell.
In the nervous system, a synapse is a structure that permits the axon of a neuron to pass an electrical or chemical signal the axon of another neuron or to another cell type.
The neuron conducting impulses towards the synapse is called pre-synaptic neuron. The neuron transmitting the electrical impulse away from the synapse is called post-synaptic neuron, if the post-synaptic cell is not neuronal it is sometimes referred to as an effector cell.
Synapses can be classified by the type of cellular structures serving as the pre- and post-synaptic components. The vast majority of synapses in the mammalian nervous system are axo-axonal (axon synapsing with another axon) or axo-dendritic synapses (axon synapsing upon a dendrite). However, a variety of other arrangements exist.
Chemical and Electrical Synapses
Chemical Synapse: A: Pre-synaptic neuron B: Post-synaptic-neuron. Neurotransmitters are stored in synaptic vesicles within the pre-synaptic neuron (2). Upon receipt of an action-potential the neurotransmitter is secreted into the synaptic cleft (3 & 4) and binds to the receptors on the post-synaptic neuron (5).
There are two varieties of synapses: electrical and chemical synapse.
In a chemical synapse, the plasma membrane of the pre-synaptic neuron is closely associated with the plasma membrane of the post-synaptic cell, with the gap between termed the synaptic cleft. The synapse is stabilized by the expression of synaptic adhesion molecules projecting from both the pre- and post-synaptic cells maintaining the close association.
Upon arrival of an action potential at the pre-synaptic axon neurotransmitters are released into the synaptic cleft via the action of voltage-gated calcium channels. This neurotransmitter binds to receptors located in the plasma membrane of the post-synaptic cell which can elicit an electrical response or the activation of a secondary messenger pathway. Because of the complexity of receptor signal transduction, chemical synapses can have complex effects on the post-synaptic cell, and are able to induce effects such as gain, or amplification, whereby the strength of the signal is increased in the post-synaptic cell.
In an electrical synapse, the pre-synaptic and post-synaptic cell membranes are fused and connected by special channels called gap junctions that are capable of passing electrical current. These gap junctions contain connexion proteins which allow ions and small molecules to flow directly from one neuron to the next. The neurons are electrically coupled and transmission across these synapses is very rapid, allowing for faster signal processing than chemical synapses. However, due to their nature electical synapses cannot induce gain of signal strength.
Electrical Synapse: The membranes of pre and post-synaptic cells are fused and punctured by gap-junctions. When open they allow the rapid diffusion of ions across the plasma membranes allowing for rapid, continuous signal processing across the synapse.
Postsynaptic Potentials and Their Integration at the Synapse
Postsynaptic potentials are excitatory or inhibitory changes in the graded membrane potential in the postsynaptic terminal of a chemical synapse.
Learning Objectives
Describe the role of postsynaptic potentials
Key Takeaways
Key Points
- Postsynaptic potentials are graded changes in the membrane potential of a postsynaptic synapse.
- Postsynaptic potentials are graded potentials and should not be confused with action potentials, although their function is to initiate or inhibit action potentials.
- Excitatory postsynaptic potentials (EPSP) bring the neuron’s potential closer to its firing threshold.
- Inhibitory postsynaptic potentials (IPSP) change the charge across the membrane to be further from the firing threshold.
- Postsynaptic potentials are subject to spatial and temporal summation.
Key Terms
- Postsynaptic potentials: Changes in the membrane potential of the postsynaptic terminal of a chemical synapse.
- inhibitory postsynaptic potential: This occurs when the opening of the ion channels results in a net gain of negative charge, the potential moves further from zero and is referred to as hyperpolarization.
- excitatory postsynaptic potential: A net depolarization that brings the neuron’s potential closer to its firing threshold.
Postsynaptic potentials are changes in the membrane potential of the postsynaptic terminal of a chemical synapse. Postsynaptic potentials are graded potentials and should not be confused with action potentials, although their function is to initiate or inhibit action potentials.
Many postsynaptic membrane receptors at chemical synapses are specialized to open ion channels. This converts a chemical signal into an electrical signal. Chemical synapses are either excitatory or inhibitory depending on how they affect the membrane potential of the postsynaptic neuron. The neurotransmitters bind to receptors on the postsynaptic terminal resulting in an opening of ion channels. At excitatory synapses, neurotransmitter binding depolarizes the postsynaptic membrane. Unlike the action potential in axonal membranes, chemically-gated ion channels open on postsynaptic membranes. Sodium and potassium diffuse simultaneously but in opposite directions.
Since the electrochemical gradient of sodium is steeper than that of potassium, a net depolarization occurs. If enough neurotransmitter binds, depolarization of the postsynaptic membrane can reach 0mV, which is higher than threshold of -30-50mV. This is an excitatory postsynaptic potential (EPSP) as it brings the neuron’s potential closer to its firing threshold (about -50mV).
Neurotransmitter binding at inhibitory synapses reduces a postsynaptic neuron’s ability to generate an action potential. Most inhibitory neurotransmitters hyperbolize the postsynaptic membrane by making it more permeable to potassium or chloride. When the opening of the ion channels results in a net gain of negative charge, the potential moves further from zero and is referred to as hyperpolarization. This is an inhibitory postsynaptic potential (IPSP).
EPSPs and IPSPs are transient changes in the membrane potential. A single EPSP at one synapse is generally far too small to trigger an action potential in the postsynaptic neuron. Postsynaptic potentials are subject to spatial and temporal summation.
Temporal summation: This figure depicts the mechanism of temporal summation in which multiple action potentials in the presynaptic cell cause a threshold depolarization in the postsynaptic cell.
Synaptic Transmission
Synaptic transmission is a chemical event which is involved in the transmission of the impulse via release, diffusion, receptor binding of neurotransmitter molecules and unidirectional communication between neurons.
Learning Objectives
Describe the process of synaptic transmission
Key Takeaways
Key Points
- In a chemical synapse, the pre and post synaptic membranes are separated by a synaptic cleft, a fluid filled space.
- The chemical event is involved in the transmission of the impulse via release, diffusion, receptor binding of neurotransmitter molecules and unidirectional communication between neurons.
- The neurotransmitter termination can occur in three ways – reuptake, enzymatic degradation in the cleft and diffusion.
In a chemical synapse, the pre and post synaptic membranes are separated by a synaptic cleft, a fluid filled space. The chemical event is involved in the transmission of the impulse via release, diffusion, receptor binding of neurotransmitter molecules and unidirectional communication between neurons.
Chemical Synapse
Synaptic vesicles inside a neuron: This pseudocolored image taken with a scanning electron microscope shows an axon terminal that was broken open to reveal synaptic vesicles (blue and orange) inside the neuron.
Neurotransmission at a chemical synapse begins with the arrival of an action potential at the presynaptic axon terminal. When an action potential reaches the axon terminal, it depolarizes the membrane and opens voltage-gated Na+ channels. Na+ ions enter the cell, further depolarizing the presynaptic membrane. This depolarization causes voltage-gated Ca2+ channels to open. Calcium ions entering the cell initiate a signaling cascade. A calcium sensing protein binds calcium and interacts with SNARE proteins. These SNARE proteins are involved in the membrane fusion. The synaptic vesicles fuse with the presynaptic axon terminal membrane and empty their contents by exocytosis into the synaptic cleft. Calcium is quickly removed from the terminal.
Fusion of a vesicle with the presynaptic membrane causes neurotransmitters to be released into the synaptic cleft. The neurotransmitter diffuses across the synaptic cleft, binding to receptor proteins on the postsynaptic membrane.
Communication at a chemical synapse: Communication at chemical synapses requires release of neurotransmitters. When the presynaptic membrane is depolarized, voltage-gated Ca2+ channels open and allow Ca2+ to enter the cell. The calcium entry causes synaptic vesicles to fuse with the membrane and release neurotransmitter molecules into the synaptic cleft. The neurotransmitter diffuses across the synaptic cleft and binds to ligand-gated ion channels in the postsynaptic membrane, resulting in a localized depolarization or hyperpolarization of the postsynaptic neuron.
The binding of a specific neurotransmitter causes particular ion channels, in this case ligand-gated channels, on the postsynaptic membrane to open. The binding of a neurotransmitter to its receptor is reversible. As long as it is bound to a post synaptic receptor, a neurotransmitter continues to affect membrane potential. The effects of the neurotransmitter generally lasts few milliseconds before being terminated. The neurotransmitter termination can occur in three ways. First, reuptake by astrocytes or presynaptic terminal where the neurotransmitter is stored or destroyed by enzymes. Second, degradation by enzymes in the synaptic cleft such as acetylcholinesterase. Third, diffusion of the neurotransmitter as it moves away from the synapse.
Types of Neurotransmitters by Function
Neurotransmitters are endogenous chemicals that transmit signals from a neuron to a target cell across a synapse.
Learning Objectives
Describe types of neurotransmitters by their function
Key Takeaways
Key Points
- Neurotransmitters are endogenous chemicals that transmit signals from a neuron to a target cell across a synapse.
- Release of neurotransmitters usually follows arrival of an action potential at the synapse, but may also follow a graded electrical potential.
- The major types of neurotransmitters include acetylcholine, biogenic amines, and amino acids.
- Biogenic amines include the catecholamines such as dopamine, norepinephrine (NE), and epinephrine, as well as indolamines such as serotonin and histamine.
Key Terms
- neurotransmitter: An endogenous chemical that transmits signals from a neuron to a target cell across a synapse.
- acetylcholine: This neurotransmitter acts on the neuromuscular juncture and is
synthesized from acetic acid and choline. - Biogenic amines: Neurotransmitters distributed in the brain, where they play a role in emotional behavior and help in regulating the biological clock.
- glutamate: An amino acid that
promotes excitatory effects by increasing the probability that the target cell will fire an action potential.
Neurotransmitters are endogenous chemicals that transmit signals from a neuron to a target cell across a synapse. Although some neurons produce and release only one kind of neurotransmitter, most make two or more and may release one or more at any given time. The coexistence of more than one neurotransmitter in the synapse makes it possible for the cell to exert several influences at the same time.
Neurotransmitter Structure
Major elements in neuron-to-neuron communication: Chemical synapses are specialized junctions through which neurons signal to each other and to non-neuronal cells such as those in muscles or glands.
Neurotransmitters are packaged into synaptic vesicles clustered beneath the membrane in the axon terminal on the presynaptic side of a synapse. They are released into and diffuse across the synaptic cleft, where they bind to specific receptors in the membrane on the postsynaptic side of the synapse. Release of neurotransmitters usually follows arrival of an action potential at the synapse, but may also follow a graded electrical potential. Low-level baseline release also occurs without electrical stimulation.
Neurotransmitters are synthesized from plentiful and simple precursors such as amino acids, which are readily available from the diet and require only a small number of biosynthetic steps to convert.
Classification of Neurotransmitters
Neurotransmitters fall into several chemical classes based on the molecular structure. The major types of neurotransmitters include acetylcholine, biogenic amines, and amino acids. The neurotransmitters can also be classified based on function (excitatory or inhibitory) and action (direct or neuromodulatory).
Acetylcholine
Acetylcholine, which acts on the neuromuscular junction, was the first neurotransmitter identified. It is synthesized from acetic acid and choline. Once released, acetylcholine binds to post-synaptic receptors and is degraded by acetylcholinesterase. Acetylcholine-releasing neurons are also found in the central nervous system (CNS).
Biogenic Amines
Biogenic amines include the catecholamines, such as dopamine, norepinephrine (NE), and epinephrine, as well as indolamines such as serotonin and histamine. Dopamine and NE are synthesized from amino acid tyrosine. Serotonin is synthesized from tryptophan. Histamine is synthesized from amino acid
histidine.
Biogenic amines are distributed in the brain, where they play a role in emotional behavior and help in regulating the biological clock. Additionally, some motor neurons of the ANS release catecholamines like NE. NE, dopamine, and histamine can be excitatory or inhibitory depending on the receptor type. Addictive drugs such as cocaine and amphetamine exert their effects primarily on the dopamine system, while addictive opiates and functional analogs of opioid peptides which regulate dopamine levels.
Amino Acids
Glutamate and gamma-aminobutyric acid (GABA) are amino acid-based neurotransmitters. The most prevalent transmitter in the human brain is glutamate, which promotes excitatory effects by increasing the probability that the target cell will fire an action potential. The next most prevalent is GABA, which is inhibitory at more than 90% of the synapses that do not use glutamate.
Neuropeptides such as Substance P and endorphins are strings of amino acids that are important in the mediation of pain signals. Enkephalin activity increases dramatically in pregnant women in labor. Endorphins are released in the so called “runner’s high.” There is also evidence that neuropeptides such as gut-brain peptides are produced by non-neural tissues in the gastrointestinal tract.
Ionotropic and Metabotropic Receptors
Although both ionotropic and metabotropic receptors are activated by neurotransmitters, ionotropic receptors are channel-linked while metabotropic receptors initiate a cascade of molecules via G-proteins.
Learning Objectives
Distinguish between ionotropic and metabotropic receptors
Key Takeaways
Key Points
- Two types of membrane bound receptors (ionotropic and metabotropic) are activated with the binding of neurotransmitters.
- Ionotropic receptors such as nicotinic acetylcholine are a group of transmembrane ion channels that open or close in response to the binding of a chemical messenger.
- Metabotropic receptor are a type of G protein -coupled receptor
- When a metabotropic receptor is activated, a series of intracellular events are triggered that can also result in ion channels opening but must involve a range of second messenger chemicals.
Key Terms
- nicotinic acetylcholine receptor: A pentamer of protein subunits with two binding sites for acetylcholine which, when bound, alter the receptor’s configuration and cause an internal pore to open.
- Ionotropic receptors: A group of transmembrane ion channels that open or close in response to the binding of a chemical messenger (ligand) such as a neurotransmitter.
- metabotropic receptors: A subtype of membrane receptors that do not form an ion channel pore but use signal transduction mechanisms, often G proteins, to activate a series of intracellular events using second messenger chemicals.
Two types of membrane-bound receptors are activated with the binding of neurotransmitters: ligand-gated ion channels (LGICs) inotropic receptors and metabotropic G- protein coupled receptors.
Ionotropic Receptors
Ionotropic receptors are a group of transmembrane ion channels that open or close in response to the binding of a chemical messenger (ligand) such as a neurotransmitter.The binding site of endogenous ligands on LGICs protein complexes are normally located on a different portion of the protein (an allosteric binding site) than the location of the ion conduction pore.The ion channel is regulated by a ligand and is usually very selective to one or more ions such as Na+, K+, Ca2+, or Cl–.
The prototypic ligand-gated ion channel is the nicotinic acetylcholine receptor. It consists of a pentamer of protein subunits with two binding sites for acetylcholine which, when bound, alter the receptor’s configuration and cause an internal pore to open. This pore allows Na+ ions to flow down their electrochemical gradient into the cell. With a sufficient number of channels opening at once, the inward flow of positive charges carried by Na+ ions depolarizes the postsynaptic membrane enough to initiate an action potential.
Metabotropic Receptors
Metabotropic receptors are a subtype of membrane receptors that do not form an ion channel pore but use signal transduction mechanisms, often G proteins, to activate a series of intracellular events using second messenger chemicals. Examples of metabotropic receptors include glutamate receptors, muscarinic acetylcholine receptors, GABAB receptors, most serotonin receptors, and receptors for norepinephrine, epinephrine, histamine, dopamine, neuropeptides, and endocannabinoids.
The metabotropic G protein-coupled receptors have seven hydrophobic transmembrane domains. When the neurotransmitter binds to the receptor, there is an activation via the G-protein that later activates the secondary messengers. Metabotropic receptors on the presynaptic membrane can inhibit or more rarely facilitate neurotransmitter release from the presynaptic neuron.
Since opening channels by metabotropic receptors involves activating a number of molecules in the intracellular mechanism, these receptors take longer to open than the inotropic receptors. They have a much longer effect than ionotropic receptors, which open quickly but only remain open for a few milliseconds. While ionotropic channels have an effect only in the immediate region of the receptor, the effects of metabotropic receptors can be more widespread throughout the cell.
Serial and Parallel Processing
Serial memory processing compares a memory to a target stimulus, while parallel processing carries out multiple operations simultaneously.
Learning Objectives
Distinguish between serial and parallel processing by nervous tissue
Key Takeaways
Key Points
- Serial memory processing can be either self-terminating or exhaustive.
- Self-terminating serial memory processing implies that comparisons stop abruptly as soon as the target is found, and then the response is generated.
- Exhaustive serial memory processing implies that comparisons continue until the entire set is compared and then a response is generated.
- Parallel processing is the ability of the brain to simultaneously process incoming stimuli of differing quality.
- Parallel processing becomes most important in vision, as the brain divides what it sees into four components: color, motion, shape, and depth.
- Serial memory processing uses internal representations of the memory set in order to compare them to a target stimulus or item.
Key Terms
- Serial memory processing: The act of attending to and processing one item at a time.
- self-terminating: Self-terminating implies that comparisons stop abruptly as soon as the target is found, and then the response is generated.
- parallel processing: Parallel processing is the ability to carry out multiple operations or tasks simultaneously.
Serial memory processing is the act of attending to and processing one item at a time, while parallel memory processing is the act of attending to and processing all items simultaneously. In short-term memory tasks, participants are given a set of items (e.g. letters, digits) one at a time and then, after varying periods of delay, are asked for recall of the items. Participants could also be asked whether a specific target item was present in their original set. The serial order of items and the relationships between them can have varying effects on speed and accuracy of recall.
Serial Memory Processing
Serial memory processing compares internal representations of the memory set to a target stimulus or item is being presented, one at a time. Reaction time increases linearly with the set size, which means the more items in the memory set, the longer it will take to compare.
Serial memory processing can be either self-terminating or exhaustive.
Self-Terminating Processing
Self-Terminating Search: This line graph depicts both positive and negative self-terminating search, comparing length of list to mean reaction time recall.
Self-terminating implies that comparisons stop abruptly as soon as the target is found, and then the response is generated. Evidence for this method is found in reaction time studies. If the reaction time slope for a positive trial (where the target was present in the memory set) is about half of the slope for a negative trial (where the target was not present in the memory set), this demonstrates self-terminating processing. This is because on average, participants (on positive trials) will stop comparisons about halfway through when they find the target match, but participants (on negative trials) must compare until the end when no target match is found.
Exhaustive Processing
On the other hand, exhaustive implies that comparisons continue until the entire set is compared and then a response is generated. Evidence for this method is also found in reaction time studies. In this case, the reaction time slope is equal for both positive and negative trials, as comparisons are made to the end in both cases. Participants may process some serial memory sets using the self-terminating method and others with the exhaustive method. There is no clear distinction about which method is better to use.
Parallel Processing
Parallel processing is the ability to carry out multiple operations or tasks simultaneously. The term is used in the contexts of human cognition, particularly in the ability of the brain to simultaneously process incoming stimuli, and in parallel computing by machines.
In parallel processing, the brain simultaneously processes incoming stimuli of differing quality. This is most important in vision, as the brain divides what it sees into four components: color, motion, shape, and depth. These are individually analyzed and then compared to stored memories, which helps the brain identify what you are viewing. The brain then combines all four components into the field of view that you see and comprehend. This is a continual and seamless operation.