77 Motor Pathways
Organization of Motor Neuron Pathways
The motor system is the part of the central nervous system that is involved with movement.
Learning Objectives
Describe the organization of motor neuron pathways
Key Takeaways
Key Points
- The pyramidal tract, which includes both the corticospinal and corticobulbar tracts, serves as the motor pathway for upper motor neuronal signals coming from the cerebral cortex and from primitive brainstem motor nuclei.
- Peripheral motor nerves carry the motor impulses from the spinal cord to the voluntary muscles.
- The large majority (90%) of motor neurons cross (decussate) to the contralateral side of the brain at the level of the brainstem.
Key Terms
- extrapyramidal system: A biological neural network that is part of the motor system that causes involuntary movements.
- corticospinal tract: The nervous system tract that conducts impulses from the brain to the spinal cord. It contains mostly motor axons and is made up of two separate tracts in the spinal cord: the lateral corticospinal tract and the anterior corticospinal tract.
- motor system: The part of the central nervous system that is involved with movement. It consists of the pyramidal and extrapyramidal systems.
- cerebral cortex: The gray, folded, outermost layer of the cerebrum that is responsible for higher brain processes such as sensation, voluntary muscle movement, thought, reasoning, and memory.
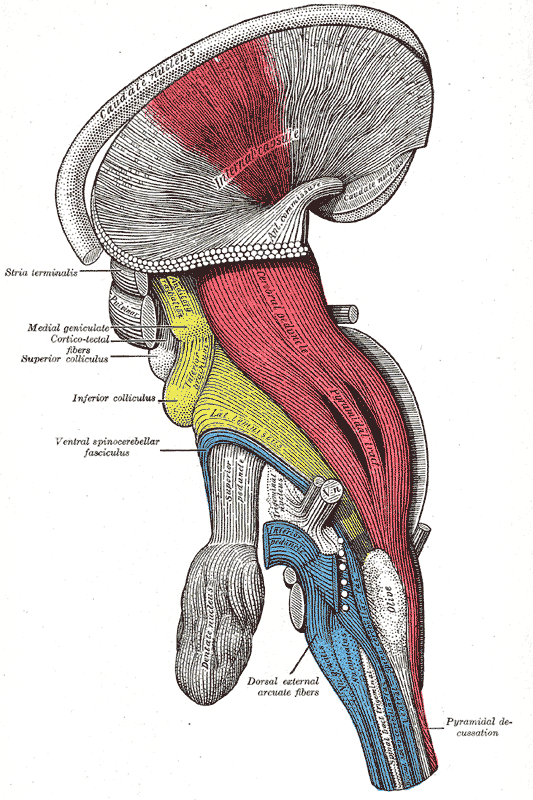
Decussation of the pyramids: A deep dissection, lateral view of a brainstem. The pyramidal tract is visible in red, and pyramidal decussation is labeled at lower right.
The motor system is the part of the central nervous system that is involved with movement. It consists of the pyramidal and extrapyramidal system.
The motor pathway, also called the pyramidal tract or the corticospinal tract, serves as the motor pathway for upper motor neuronal signals coming from the cerebral cortex and from primitive brainstem motor nuclei. There are upper and lower motor neurons in the corticospinal tract.
The motor impulses originate in the giant pyramidal cells (Betz cells) of the motor area, i.e., the precentral gyrus of the cerebral cortex. These are the upper motor neurons of the corticospinal tract. The axons of these cells pass from the cerebral cortex to the midbrain and the medulla oblongata. Peripheral motor nerves carry the motor impulses from the anterior horn to the voluntary muscles.
Cortical upper motor neurons originate from Brodmann areas 1, 2, 3, 4, and 6, then descend into the posterior limb of the internal capsule, through the crus cerebri, down through the pons, and to the medullary pyramids, where about 90% of the axons cross to the contralateral side at the decussation of the pyramids. They then descend as the lateral corticospinal tract.
These axons synapse with lower motor neurons in the ventral horns of all levels of the spinal cord. The remaining 10% of axons descend on the ipsilateral side as the ventral corticospinal tract. These axons also synapse with lower motor neurons in the ventral horns. Most of them will cross to the contralateral side of the cord (via the anterior white commissure) just before synapsing.
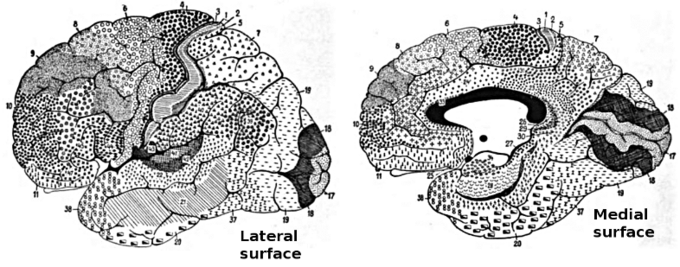
Brodmann areas of the brain: This drawing shows the regions of the human cerebral cortex as delineated by Korvinian Brodmann on the basis of cytoarchitecture.
The midbrain nuclei include four motor tracts that send upper motor neuronal axons down the spinal cord to lower motor neurons. These are the rubrospinal tract, the vestibulospinal tract, the tectospinal tract, and the reticulospinal tract.
The function of lower motor neurons can be divided into two different groups: the lateral corticospinal tract and the anterior corticalspinal tract. The lateral tract contains upper motor neuronal axons that synapse on the dorsal lateral lower motor neurons, which are involved in distal limb control.
The anterior corticospinal tract descends ipsilaterally in the anterior column, where the axons emerge and either synapse on ventromedial lower motor neurons in the ventral horn ipsilaterally or descussate at the anterior white commissure where they synapse on ventromedial lower motor neurons contralaterally.
The ventromedial lower motor neurons control the large, postural muscles of the axial skeleton. These lower motor neurons, unlike those of the dorsal lateral, are located in the ventral horn throughout the spinal cord.
Spinal cord tracts: This diagram of spinal cord tracts shows the motor and efferent pathways in red and the sensory and afferent pathways in blue. Included in the diagram are the following motor pathways: corticospinal tracts (pyramidal tract), and extrapyramidal tracts (tectospinal tract not delineated).
The Role of the Basal Ganglia in Movement
The basal ganglia are responsible for voluntary motor control, procedural learning, and eye movement, as well as cognitive and emotional functions.
Learning Objectives
Describe the role of the basal ganglia in movement
Key Takeaways
Key Points
- The basal ganglia are studied extensively in the context of two disorders of the basal ganglia: Parksinson’s disease and Huntington’s disease.
- Hemiballismus, a movement disorder arising from neuronal damage in the subthalamic nucleus, presents with violent movements of the arms and legs.
- Eye movement, a function of the basal ganglia, is influenced by the superior colliculus, a region of the brain that directs eye movement to specific points in space in response to stimuli.
- Basal ganglia are also thought to play a role in motivation.
- In the basal ganglia,
the majority of the neurons uses gamma-aminobutyric acid (GABA) as the neurotransmitter
and have inhibitory effects on their
targets.
Key Terms
- hemiballismus: A rare movement disorder with involuntary flinging motions of the extremities.
- voluntary motor control: The act of directing motion with intent.
- forebrain: The anterior part of the brain, including the cerebrum, thalamus, and hypothalamus.
- nucleus accumbens: A region in the basal forebrain rostral to the preoptic area of the hypothalamus. This region and the olfactory tubercle collectively form the ventral striatum.
Location of the Basal Ganglia
The basal ganglia (or basal nuclei) are a group of nuclei of varied origin in the brains of vertebrates that act as a cohesive functional unit. They are situated at the base of the forebrain and are strongly connected with the cerebral cortex, thalamus, and other brain areas.
The basal ganglia are associated with a variety of functions, including voluntary motor control, procedural learning relating to routine behaviors or habits such as bruxism and eye movements, as well as cognitive and emotional functions.
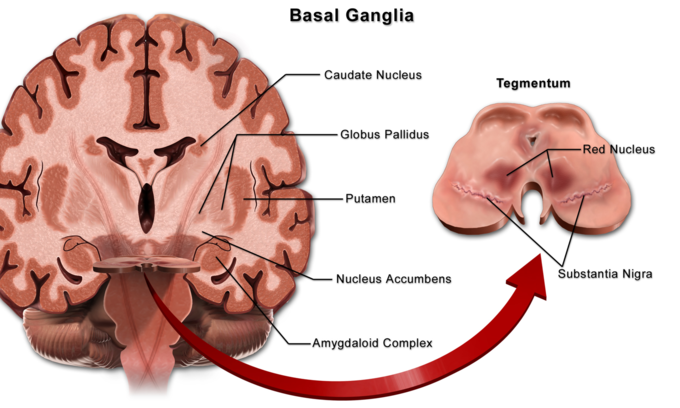
Basal ganglia: Locations of the basal ganglia.
Action Selection
Currently popular theories hold that the basal ganglia play a primary role in action selection. Action selection is the decision of which of several possible behaviors to execute at a given time.
Experimental studies show that the basal ganglia exert an inhibitory influence on a number of motor systems, and that a release of this inhibition permits a motor system to become active. The behavior switching that takes place within the basal ganglia is influenced by signals from many parts of the brain, including the prefrontal cortex, which plays a key role in executive functions.
Movement
The greatest source of insight into the functions of the basal ganglia has come from the study of two neurological disorders, Parkinson’s disease and Huntington’s disease. For both of these disorders, the nature of the neural damage is well-understood and can be correlated with the resulting symptoms.
Parkinson’s disease involves the major loss of dopaminergic cells in the substantia nigra. Huntington’s disease involves the massive loss of medium spiny neurons in the striatum.
The symptoms of the two diseases are virtually opposite: Parkinson’s disease is characterized by a gradual loss of the ability to initiate movement, whereas Huntington’s disease is characterized by an inability to prevent parts of the body from moving unintentionally.
It is noteworthy that, although both diseases have cognitive symptoms, especially in their advanced stages, the most salient symptoms relate to the ability to initiate and control movement. Thus, both are classified primarily as movement disorders.
A different movement disorder, called hemiballismus, may result from damage restricted to the subthalamic nucleus. Hemiballismus is characterized by violent and uncontrollable flinging movements of the arms and legs.
Function in Eye Movement
One of the most intensively studied functions of the basal ganglia is their role in controlling eye movements. Eye movement is influenced by an extensive network of brain regions that converge on a midbrain area called the superior colliculus (SC).
The SC is a layered structure whose layers form two-dimensional retinotopic maps of visual space. A bump of neural activity in the deep layers of the SC drives eye movement toward the corresponding point in space.
Motivation
Although the role of the basal ganglia in motor control is clear, there are also many indications that it is involved in the control of behavior in a more fundamental way, at the level of motivation. In Parkinson’s disease, the ability to execute the components of movement is not greatly affected, but motivational factors such as hunger fail to cause movements to be initiated or switched at the proper times.
The immobility of patients with Parkingson’s disease has sometimes been described as a paralysis of the will. These patients have occasionally been observed to show a phenomenon called kinesia paradoxica, in which a person who is otherwise immobile responds to an emergency in a coordinated and energetic way, then lapses back into immobility once the emergency has passed.
The role in motivation of the limbic part of the basal ganglia—the nucleus accumbens (NA), ventral pallidum, and ventral tegmental area (VTA)—is particularly well established. Thousands of experimental studies combine to demonstrate that the dopaminergic projection from the VTA to the NA plays a central role in the brain’s reward system.
Numerous things that people find rewarding, including addictive drugs, good-tasting food, and sex, have been shown to elicit activation of the VTA dopamine system. Damage to the NA or VTA can produce a state of profound torpor.
Neurotransmitters
In most regions of the brain, the predominant classes of neurons use glutamate as the neurotransmitter and have excitatory effects on their targets. In the basal ganglia, however, the great majority of neurons uses gamma-aminobutyric acid (GABA) as the neurotransmitter and have inhibitory effects on their targets.
The inputs from the cortex and thalamus to the striatum and subthalamic nucleus are glutamatergic, but the outputs from the striatum, pallidum, and substantia nigra pars reticulata all use GABA. Thus, following the initial excitation of the striatum, the internal dynamics of the basal ganglia are dominated by inhibition and disinhibition.
Other neurotransmitters have important modulatory effects. Dopamine is used by the projection from the substantia nigra pars compacta to the dorsal striatum and also in the analogous projection from the ventral tegmental area to the ventral striatum (nucleus accumbens).
Acetylcholine also plays an important role, as it is used both by several external inputs to the striatum and by a group of striatal interneurons. Although cholinergic cells make up only a small fraction of the total population, the striatum has one of the highest acetylcholine concentrations of any brain structure.
Main circuits of the basal ganglia: This diagram shows the main circuits of the basal ganglia. Two coronal slices have been superimposed to include the involved basal ganglia structures. The + and – signs at the point of the arrows indicate whether the pathway is excitatory or inhibitory, respectively, in effect. Green arrows refer to excitatory glutamatergic pathways, red arrows refer to inhibitory GABAergic pathways and turquoise arrows refer to dopaminergic pathways that are excitatory on the direct pathway and inhibitory on the indirect pathway.
Modulation of Movement by the Cerebellum
The cerebellum is important for motor control—specifically coordination, precision, and timing—as well as some forms of motor learning.
Learning Objectives
Describe the role of the cerebellum in movement modulation
Key Takeaways
Key Points
- The cerebellum is a parallel grooved structure at the bottom of the brain containing a highly regular cellular arrangement of Purkinje cells, granule cells, and other cell types.
- The cerebellum adjusts to changes in sensorimotor relationships, possibly functioning as in the Marr-Albus theory: Strong inputs from a single climbing fiber serve as a teaching signal to change the strength of impulses from the corresponding group of parallel fibers.
- Four principles of cerebellum function have been identified. They include: feedforward processing, divergence and convergence, modularity, and plasticity.
Key Terms
- Purkinje cells: A class of GABAergic neurons located in the cerebellum.
- mossy fibers: One of the major inputs to the cerebellum from sources such as the cerebral cortex.
- granule cells: These cells receive excitatory input from mossy fibers that originate from pontine nuclei.
The cerebellum is a region of the brain that plays an important role in motor control. It may also be involved in some cognitive functions such as attention and language, and in regulating fear and pleasure responses, but its movement-related functions are the most solidly established. The cerebellum does not initiate movement, but it contributes to coordination, precision, and accurate timing.
It receives input from sensory systems of the spinal cord and from other parts of the brain, including the cerebral cortex, and integrates these inputs to fine-tune motor activity. Because of this fine-tuning function, damage to the cerebellum does not cause paralysis, but instead produces disorders in fine movement, equilibrium, posture, and motor learning.
The cerebellum differs from most other parts of the brain, especially the cerebral cortex, in regards to the ability of signals to move unidirectionally from input to output. This feedforward mode of operation means that the cerebellum cannot generate self-sustaining patterns of neural activity, in contrast to the cerebral cortex. However, the cerebellum can receive information from the cerebral cortex and processes this information to send motor impulses to the skeletal muscle.
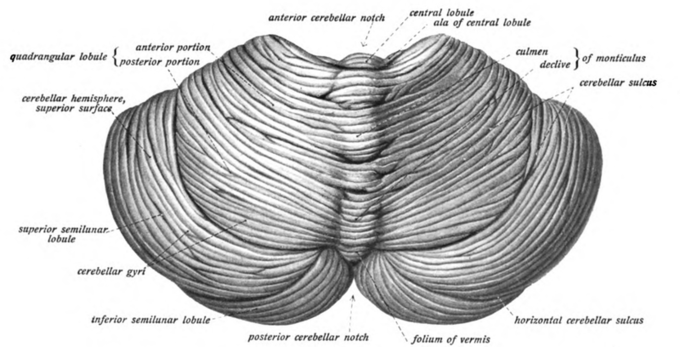
Cerebellum: View of the cerebellum from above and behind.
Anatomy of the Cerebellum
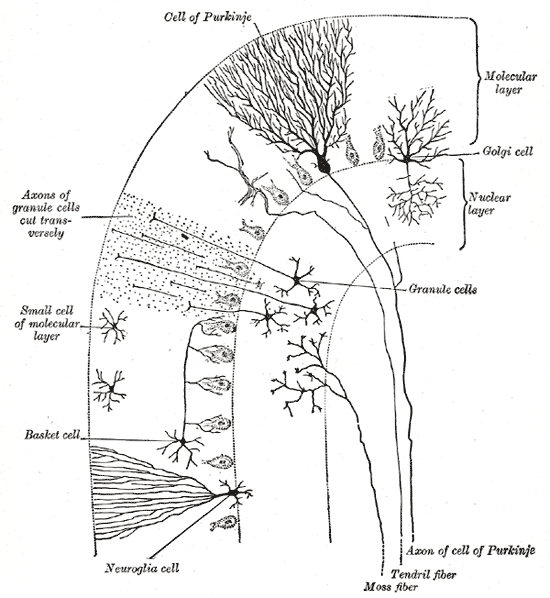
Cerebellum cells: View of the cerebellum from above and behind.
In terms of anatomy, the cerebellum has the appearance of a separate structure attached to the bottom of the brain, tucked underneath the cerebral hemispheres. The surface of the cerebellum is covered with finely spaced parallel grooves, in striking contrast to the broad irregular convolutions of the cerebral cortex. These parallel grooves conceal the fact that the cerebellum is actually a continuous thin layer of tissue (the cerebellar cortex), tightly folded in the style of an accordion.
Within this thin layer are several types of neurons with a highly regular arrangement, the most important being Purkinje cells and granule cells. This complex neural network gives rise to a massive signal-processing capability, but almost all of its output is directed to a set of small, deep cerebellar nuclei lying in the interior of the cerebellum.
Function
Marr-Albus Theory
In addition to its direct role in motor control, the cerebellum is also necessary for several types of motor learning, the most notable one being learning to adjust to changes in sensorimotor relationships.
Several theoretical models have been developed to explain sensorimotor calibration in terms of synaptic plasticity within the cerebellum. Most of them derive from early models formulated by David Marr and James Albus, which were motivated by the observation that each cerebellar Purkinje cell receives two dramatically different types of input.
It receives input from thousands of parallel fibers, each individually very weak. However, each cerebellar Purkinje cell also gets input from one single climbing fiber, which is so strong that a single climbing fiber action potential will reliably cause a target Purkinje cell to fire a burst of action potentials.
The basic concept of the Marr-Albus theory is that the climbing fiber serves as a teaching signal, which induces a long-lasting change in the strength of synchronously activated parallel fiber inputs. Observations of long-term depression in parallel fiber inputs have provided support for theories of this type, but their validity remains controversial.
Insights from Cerebellar Dysfunction
The strongest clues to the function of the cerebellum have come from examining the consequences of damage to it. Animals and humans with cerebellar dysfunction show, above all, problems with motor control. They continue to be able to generate motor activity, but it loses precision, producing erratic, uncoordinated, or incorrectly timed movements.
A standard test of cerebellar function is to reach with the tip of the finger for a target at arm’s length. A healthy person will move the fingertip in a rapid straight trajectory, whereas a person with cerebellar damage will reach slowly and erratically, with many mid-course corrections.
Deficits in non-motor functions are more difficult to detect. Thus, the general conclusion reached decades ago is that the basic function of the cerebellum is not to initiate movements, or to decide which movements to execute, but rather to calibrate the detailed form of a movement.
The comparative simplicity and regularity of the cerebellar anatomy led to an early hope that it might imply a similar simplicity of computational function. Although a full understanding of cerebellar function remains elusive, at least four principles are identified as important: 1) feedforward processing, 2) divergence and convergence, 3) modularity, and 4) plasticity.
- Feedforward processing: Refers to the unidirectional movement of signals through the system from input to output, with very little recurrent internal transmission. This means that the cerebellum, in contrast to the cerebral cortex, cannot generate self-sustaining patterns of neural activity. Signals enter the circuit, are processed by each stage in sequential order, and then leave.
- Divergence and convergence: The 1000 or so Purkinje cells belonging to a microzone may receive input from as many as 100 million parallel fibers, and focus their own output down to a group of less than 50 deep nuclear cells. Thus, the cerebellar network receives a modest number of inputs, processes them very extensively through its rigorously structured internal network, and sends out the results via a very limited number of output cells.
- Modularity: The cerebellar system is functionally divided into independent modules. All modules have a similar internal structure, but with different inputs and outputs. The output of one module does not appear to significantly influence the activity of other modules
- Plasticity: The synapses between parallel fibers and Purkinje cells, and the synapses between mossy fibers and deep nuclear cells, are both susceptible to modification of their strength. The influence of each parallel fiber on nuclear cells is adjustable. This arrangement gives tremendous flexibility for fine-tuning the relationship between the cerebellar inputs and outputs.
Functions of the Cerebellum in Integrating Movements
The cerebellum uses feedforward processing and modularity to process information.
Learning Objectives
Describe the functions of the cerebellum in integrating movments
Key Takeaways
Key Points
- The function of the cerebellum can be described by the principles of feedforward processing and modularity.
- Feedforward processing means signals move in one direction through the cerebellum, from input to output.
- Modularity describes the modular nature of the cerebellar system, where modules with similar structures function relatively independently. Modules consist of clusters of neurons with common inputs but distinct outputs.
Key Terms
- purkinje: Purkinje cells are a class of GABAergic neurons located in the cerebellar cortex. They are some of the largest neurons in the human brain, with an intricately elaborate dendritic arbor characterized by a large number of dendritic spines.
- feedforward processing: A property of some neural circuits where signals move unidirectionally through the system from input to output, with very little recurrent internal transmission.
- microzone: A group of Purkinje cells that all have the same somatotopic receptive field. Microzones contain on the order of 1,000 Purkinje cells each, arranged in a long, narrow strip, and oriented perpendicular to the cortical folds.
Cerebellar Function
Feedforward Processing
The cerebellum differs from most other parts of the brain in that the signal processing is almost entirely feedforward—that is, signals move unidirectionally through the system from input to output, with very little recurrent internal transmission.
The small amount of recurrence that does exist consists of mutual inhibition; there are no mutually excitatory circuits. This feedforward mode of operation means that the cerebellum, in contrast to the cerebral cortex, cannot generate self-sustaining patterns of neural activity.
Signals enter the circuit, are processed by each stage in sequential order, and then leave. As Eccles, Ito, and Szentágothai wrote,”This elimination in the design of all possibility of reverberatory chains of neuronal excitation is undoubtedly a great advantage in the performance of the cerebellum as a computer, because what the rest of the nervous system requires from the cerebellum is presumably not some output expressing the operation of complex reverberatory circuits in the cerebellum, but rather a quick and clear response to the input of any particular set of information.”
Divergence and Convergence
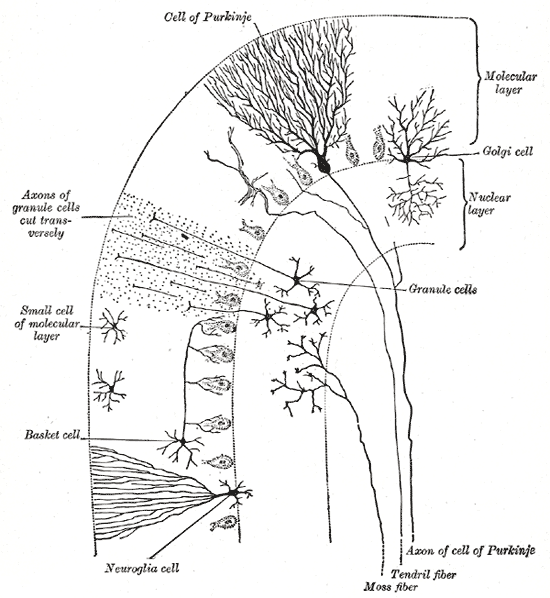
Cells of the Cerebellum: Transverse section of a cerebellar folium, showing its principal cell types and connections.
In the human cerebellum, information from 200 million mossy fiber inputs is expanded to 40 billion granule cells, whose parallel fiber outputs then converge onto 15 million Purkinje cells. Because of the way that they are lined up longitudinally, the 1,000 or so Purkinje cells belonging to a microzone may receive input from as many as 100 million parallel fibers and focus their own output down to a group of less than 50 deep nuclear cells.
Thus, the cerebellar network receives a modest number of inputs, processes them very extensively through its rigorously structured internal network, and sends out the results via a very limited number of output cells.
Modularity
The cerebellar system is functionally divided into more or less independent modules, that probably number in the hundreds to thousands. All modules have a similar internal structure, but different inputs and outputs.
A module (a multizonal microcompartment in the terminology of Apps and Garwicz) consists of a small cluster of neurons in the inferior olivary nucleus, a set of long narrow strips of Purkinje cells in the cerebellar cortex (microzones), and a small cluster of neurons in one of the deep cerebellar nuclei.
Different modules share input from mossy fibers and parallel fibers, but in other respects they appear to function independently. The output of one module does not appear to significantly influence the activity of other modules.
Plasticity
The synapses between parallel fibers and Purkinje cells, and the synapses between mossy fibers and deep nuclear cells, are both susceptible to modification of their strength. In a single cerebellar module, input from as many as a billion parallel fibers converge onto a group of less than 50 deep nuclear cells, and the influence of each parallel fiber on those nuclear cells is adjustable. This arrangement gives tremendous flexibility for fine-tuning the relationships between the cerebellar inputs and outputs.
Zones and microzones in the cerebellum: This schematic illustration of the structure of zones and microzones in the cerebellum shows three levels of magnification. These zones and microzones help explain the modular nature of the cerebellar function. On the left is a simplified illustration of what the cerebellar cortex would look like if all the folds were straightened out—the vertical dimension is the rostro-caudal axis of the cerebellum, the horizontal dimension is the medio-lateral axis. A zone is a longitudinally oriented strip of the cortex, and a microzone is a thin, longitudinally oriented portion of a zone. As the illustration on the right shows, Purkinje cell dendritic trees are flattened in a way that aligns with the microzone length, and parallel fibers cross the microzones at right angles.