161 Metabolic Reactions
Breakdown of Pyruvate
After glycolysis, pyruvate is converted into acetyl CoA in order to enter the citric acid cycle.
Learning Objectives
Explain why cells break down pyruvate
Key Takeaways
Key Points
- In the conversion of pyruvate to acetyl CoA, each pyruvate molecule loses one carbon atom with the release of carbon dioxide.
- During the breakdown of pyruvate, electrons are transferred to NAD+ to produce NADH, which will be used by the cell to produce ATP.
- In the final step of the breakdown of pyruvate, an acetyl group is transferred to Coenzyme A to produce acetyl CoA.
Key Terms
- acetyl CoA: a molecule that conveys the carbon atoms from glycolysis (pyruvate) to the citric acid cycle to be oxidized for energy production
Breakdown of Pyruvate
In order for pyruvate, the product of glycolysis, to enter the next pathway, it must undergo several changes to become acetyl Coenzyme A (acetyl CoA). Acetyl CoA is a molecule that is further converted to oxaloacetate, which enters the citric acid cycle (Krebs cycle). The conversion of pyruvate to acetyl CoA is a three-step process.
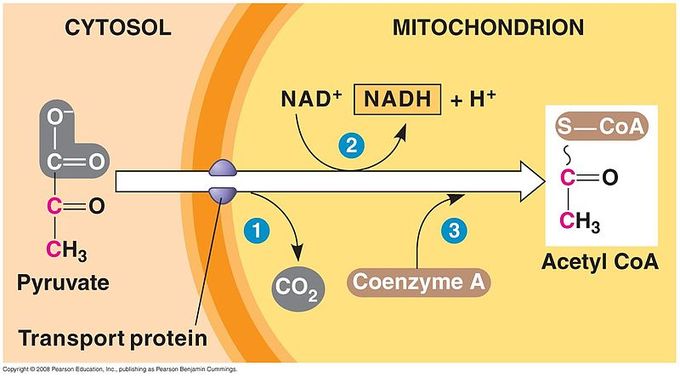
Breakdown of Pyruvate: Each pyruvate molecule loses a carboxylic group in the form of carbon dioxide. The remaining two carbons are then transferred to the enzyme CoA to produce Acetyl CoA.
Step 1. A carboxyl group is removed from pyruvate, releasing a molecule of carbon dioxide into the surrounding medium. (Note: carbon dioxide is one carbon attached to two oxygen atoms and is one of the major end products of cellular respiration. ) The result of this step is a two-carbon hydroxyethyl group bound to the enzyme pyruvate dehydrogenase; the lost carbon dioxide is the first of the six carbons from the original glucose molecule to be removed. This step proceeds twice for every molecule of glucose metabolized (remember: there are two pyruvate molecules produced at the end of glycolysis); thus, two of the six carbons will have been removed at the end of both of these steps.
Step 2. The hydroxyethyl group is oxidized to an acetyl group, and the electrons are picked up by NAD+, forming NADH (the reduced form of NAD+). The high- energy electrons from NADH will be used later by the cell to generate ATP for energy.
Step 3. The enzyme-bound acetyl group is transferred to CoA, producing a molecule of acetyl CoA. This molecule of acetyl CoA is then further converted to be used in the next pathway of metabolism, the citric acid cycle.
Citric Acid Cycle
The citric acid cycle is a series of reactions that produces two carbon dioxide molecules, one GTP/ATP, and reduced forms of NADH and FADH2.
Learning Objectives
List the steps of the Krebs (or citric acid) cycle
Key Takeaways
Key Points
- The four-carbon molecule, oxaloacetate, that began the cycle is regenerated after the eight steps of the citric acid cycle.
- The eight steps of the citric acid cycle are a series of redox, dehydration, hydration, and decarboxylation reactions.
- Each turn of the cycle forms one GTP or ATP as well as three NADH molecules and one FADH2 molecule, which will be used in further steps of cellular respiration to produce ATP for the cell.
Key Terms
- citric acid cycle: a series of chemical reactions used by all aerobic organisms to generate energy through the oxidization of acetate derived from carbohydrates, fats, and proteins into carbon dioxide
- Krebs cycle: a series of enzymatic reactions that occurs in all aerobic organisms; it involves the oxidative metabolism of acetyl units and serves as the main source of cellular energy
- mitochondria: in cell biology, a mitochondrion (plural mitochondria) is a membrane-enclosed organelle, often described as “cellular power plants” because they generate most of the ATP
Citric Acid Cycle (Krebs Cycle)
Like the conversion of pyruvate to acetyl CoA, the citric acid cycle takes place in the matrix of the mitochondria. Almost all of the enzymes of the citric acid cycle are soluble, with the single exception of the enzyme succinate dehydrogenase, which is embedded in the inner membrane of the mitochondrion. Unlike glycolysis, the citric acid cycle is a closed loop: the last part of the pathway regenerates the compound used in the first step. The eight steps of the cycle are a series of redox, dehydration, hydration, and decarboxylation reactions that produce two carbon dioxide molecules, one GTP/ATP, and reduced forms of NADH and FADH2. This is considered an aerobic pathway because the NADH and FADH2 produced must transfer their electrons to the next pathway in the system, which will use oxygen. If this transfer does not occur, the oxidation steps of the citric acid cycle also do not occur. Note that the citric acid cycle produces very little ATP directly and does not directly consume oxygen.
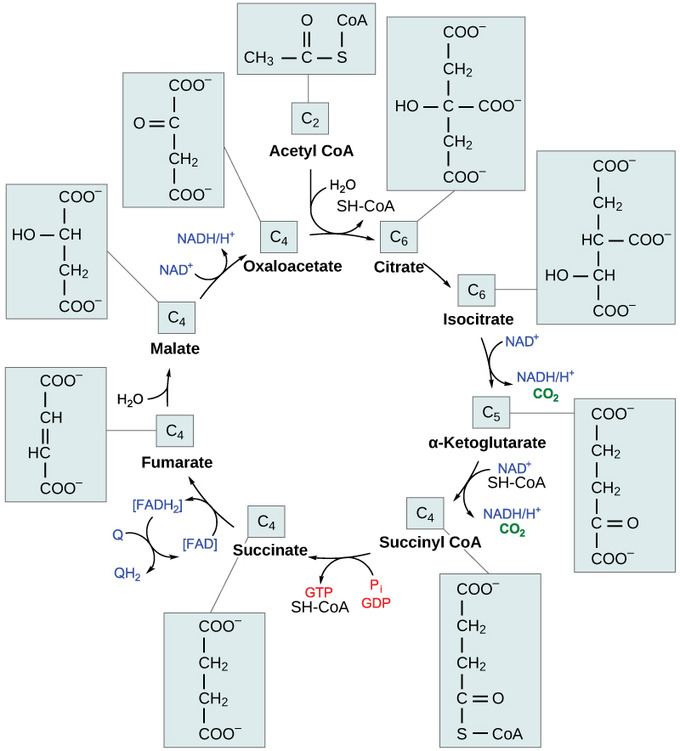
The citric acid cycle: In the citric acid cycle, the acetyl group from acetyl CoA is attached to a four-carbon oxaloacetate molecule to form a six-carbon citrate molecule. Through a series of steps, citrate is oxidized, releasing two carbon dioxide molecules for each acetyl group fed into the cycle. In the process, three NAD+ molecules are reduced to NADH, one FAD molecule is reduced to FADH2, and one ATP or GTP (depending on the cell type) is produced (by substrate-level phosphorylation). Because the final product of the citric acid cycle is also the first reactant, the cycle runs continuously in the presence of sufficient reactants.
Steps in the Citric Acid Cycle
Step 1. The first step is a condensation step, combining the two-carbon acetyl group (from acetyl CoA) with a four-carbon oxaloacetate molecule to form a six-carbon molecule of citrate. CoA is bound to a sulfhydryl group (-SH) and diffuses away to eventually combine with another acetyl group. This step is irreversible because it is highly exergonic. The rate of this reaction is controlled by negative feedback and the amount of ATP available. If ATP levels increase, the rate of this reaction decreases. If ATP is in short supply, the rate increases.
Step 2. Citrate loses one water molecule and gains another as citrate is converted into its isomer, isocitrate.
Steps 3 and 4. In step three, isocitrate is oxidized, producing a five-carbon molecule, α-ketoglutarate, together with a molecule of CO2 and two electrons, which reduce NAD+ to NADH. This step is also regulated by negative feedback from ATP and NADH and by a positive effect of ADP. Steps three and four are both oxidation and decarboxylation steps, which release electrons that reduce NAD+ to NADH and release carboxyl groups that form CO2 molecules. α-Ketoglutarate is the product of step three, and a succinyl group is the product of step four. CoA binds the succinyl group to form succinyl CoA. The enzyme that catalyzes step four is regulated by feedback inhibition of ATP, succinyl CoA, and NADH.
Step 5. A phosphate group is substituted for coenzyme A, and a high- energy bond is formed. This energy is used in substrate-level phosphorylation (during the conversion of the succinyl group to succinate) to form either guanine triphosphate (GTP) or ATP. There are two forms of the enzyme, called isoenzymes, for this step, depending upon the type of animal tissue in which they are found. One form is found in tissues that use large amounts of ATP, such as heart and skeletal muscle. This form produces ATP. The second form of the enzyme is found in tissues that have a high number of anabolic pathways, such as liver. This form produces GTP. GTP is energetically equivalent to ATP; however, its use is more restricted. In particular, protein synthesis primarily uses GTP.
Step 6. Step six is a dehydration process that converts succinate into fumarate. Two hydrogen atoms are transferred to FAD, producing FADH2. The energy contained in the electrons of these atoms is insufficient to reduce NAD+ but adequate to reduce FAD. Unlike NADH, this carrier remains attached to the enzyme and transfers the electrons to the electron transport chain directly. This process is made possible by the localization of the enzyme catalyzing this step inside the inner membrane of the mitochondrion.
Step 7. Water is added to fumarate during step seven, and malate is produced. The last step in the citric acid cycle regenerates oxaloacetate by oxidizing malate. Another molecule of NADH is produced.
Products of the Citric Acid Cycle
Two carbon atoms come into the citric acid cycle from each acetyl group, representing four out of the six carbons of one glucose molecule. Two carbon dioxide molecules are released on each turn of the cycle; however, these do not necessarily contain the most recently-added carbon atoms. The two acetyl carbon atoms will eventually be released on later turns of the cycle; thus, all six carbon atoms from the original glucose molecule are eventually incorporated into carbon dioxide. Each turn of the cycle forms three NADH molecules and one FADH2 molecule. These carriers will connect with the last portion of aerobic respiration to produce ATP molecules. One GTP or ATP is also made in each cycle. Several of the intermediate compounds in the citric acid cycle can be used in synthesizing non-essential amino acids; therefore, the cycle is amphibolic (both catabolic and anabolic).
Importance of Glycolysis
Glycolysis is the first step in the breakdown of glucose to extract energy for cellular metabolism.
Learning Objectives
Explain the importance of glycolysis to cells
Key Takeaways
Key Points
- Glycolysis is present in nearly all living organisms.
- Glucose is the source of almost all energy used by cells.
- Overall, glycolysis produces two pyruvate molecules, a net gain of two ATP molecules, and two NADH molecules.
Key Terms
- glycolysis: the cellular metabolic pathway of the simple sugar glucose to yield pyruvic acid and ATP as an energy source
- heterotroph: an organism that requires an external supply of energy in the form of food, as it cannot synthesize its own
Nearly all of the energy used by living cells comes to them from the energy in the bonds of the sugar glucose. Glucose enters heterotrophic cells in two ways. One method is through secondary active transport in which the transport takes place against the glucose concentration gradient. The other mechanism uses a group of integral proteins called GLUT proteins, also known as glucose transporter proteins. These transporters assist in the facilitated diffusion of glucose. Glycolysis is the first pathway used in the breakdown of glucose to extract energy. It takes place in the cytoplasm of both prokaryotic and eukaryotic cells. It was probably one of the earliest metabolic pathways to evolve since it is used by nearly all of the organisms on earth. The process does not use oxygen and is, therefore, anaerobic.
Glycolysis is the first of the main metabolic pathways of cellular respiration to produce energy in the form of ATP. Through two distinct phases, the six-carbon ring of glucose is cleaved into two three-carbon sugars of pyruvate through a series of enzymatic reactions. The first phase of glycolysis requires energy, while the second phase completes the conversion to pyruvate and produces ATP and NADH for the cell to use for energy. Overall, the process of glycolysis produces a net gain of two pyruvate molecules, two ATP molecules, and two NADH molecules for the cell to use for energy. Following the conversion of glucose to pyruvate, the glycolytic pathway is linked to the Krebs Cycle, where further ATP will be produced for the cell’s energy needs.
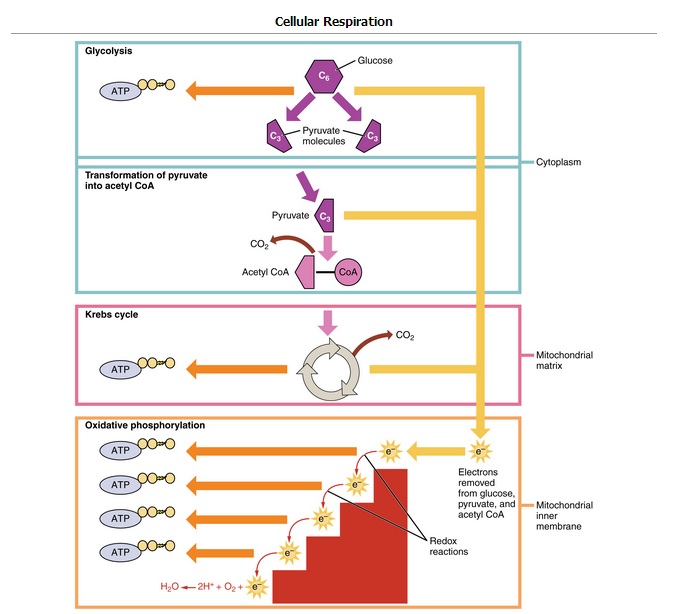
Cellular Respiration: Glycolysis is the first pathway of cellular respiration that oxidizes glucose molecules. It is followed by the Krebs cycle and oxidative phosphorylation to produce ATP.
Electron Transport Chain
The electron transport chain uses the electrons from electron carriers to create a chemical gradient that can be used to power oxidative phosphorylation.
Learning Objectives
Describe how electrons move through the electron transport chain
Key Takeaways
Key Points
- Oxidative phosphorylation is the metabolic pathway in which electrons are transferred from electron donors to electron acceptors in redox reactions; this series of reactions releases energy which is used to form ATP.
- There are four protein complexes (labeled complex I-IV) in the electron transport chain, which are involved in moving electrons from NADH and FADH2 to molecular oxygen.
- Complex I establishes the hydrogen ion gradient by pumping four hydrogen ions across the membrane from the matrix into the intermembrane space.
- Complex II receives FADH2, which bypasses complex I, and delivers electrons directly to the electron transport chain.
- Ubiquinone (Q) accepts the electrons from both complex I and complex II and delivers them to complex III.
- Complex III pumps protons through the membrane and passes its electrons to cytochrome c for transport to the fourth complex of proteins and enzymes.
- Complex IV reduces oxygen; the reduced oxygen then picks up two hydrogen ions from the surrounding medium to make water.
Key Terms
- prosthetic group: The non-protein component of a conjugated protein.
- complex: A structure consisting of a central atom, molecule, or protein weakly connected to surrounding atoms, molecules, or proteins.
- ubiquinone: A lipid soluble substance that is a component of the electron transport chain and accepts electrons from complexes I and II.
Oxidative phosphorylation is a highly efficient method of producing large amounts of ATP, the basic unit of energy for metabolic processes. During this process electrons are exchanged between molecules, which creates a chemical gradient that allows for the production of ATP. The most vital part of this process is the electron transport chain, which produces more ATP than any other part of cellular respiration.
Electron Transport Chain
The electron transport chain is the final component of aerobic respiration and is the only part of glucose metabolism that uses atmospheric oxygen. Electron transport is a series of redox reactions that resemble a relay race. Electrons are passed rapidly from one component to the next to the endpoint of the chain, where the electrons reduce molecular oxygen, producing water. This requirement for oxygen in the final stages of the chain can be seen in the overall equation for cellular respiration, which requires both glucose and oxygen.
A complex is a structure consisting of a central atom, molecule, or protein weakly connected to surrounding atoms, molecules, or proteins. The electron transport chain is an aggregation of four of these complexes (labeled I through IV), together with associated mobile electron carriers. The electron transport chain is present in multiple copies in the inner mitochondrial membrane of eukaryotes and the plasma membrane of prokaryotes.
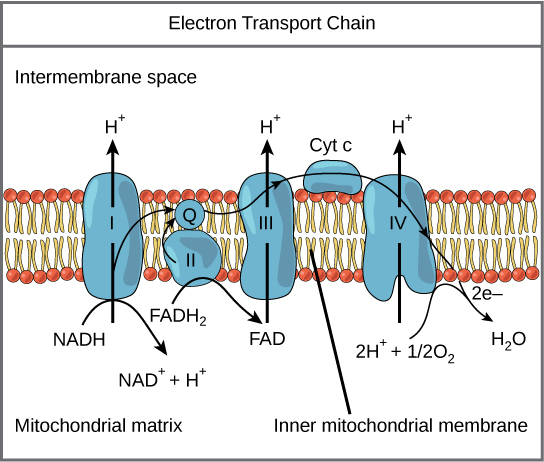
The electron transport chain: The electron transport chain is a series of electron transporters embedded in the inner mitochondrial membrane that shuttles electrons from NADH and FADH2 to molecular oxygen. In the process, protons are pumped from the mitochondrial matrix to the intermembrane space, and oxygen is reduced to form water.
Complex I
To start, two electrons are carried to the first complex aboard NADH. Complex I is composed of flavin mononucleotide (FMN) and an enzyme containing iron-sulfur (Fe-S). FMN, which is derived from vitamin B2 (also called riboflavin), is one of several prosthetic groups or co-factors in the electron transport chain. A prosthetic group is a non-protein molecule required for the activity of a protein. Prosthetic groups can be organic or inorganic and are non-peptide molecules bound to a protein that facilitate its function.
Prosthetic groups include co-enzymes, which are the prosthetic groups of enzymes. The enzyme in complex I is NADH dehydrogenase, a very large protein containing 45 amino acid chains. Complex I can pump four hydrogen ions across the membrane from the matrix into the intermembrane space; it is in this way that the hydrogen ion gradient is established and maintained between the two compartments separated by the inner mitochondrial membrane.
Q and Complex II
Complex II directly receives FADH2, which does not pass through complex I. The compound connecting the first and second complexes to the third is ubiquinone (Q). The Q molecule is lipid soluble and freely moves through the hydrophobic core of the membrane. Once it is reduced to QH2, ubiquinone delivers its electrons to the next complex in the electron transport chain. Q receives the electrons derived from NADH from complex I and the electrons derived from FADH2 from complex II, including succinate dehydrogenase. This enzyme and FADH2 form a small complex that delivers electrons directly to the electron transport chain, bypassing the first complex. Since these electrons bypass, and thus do not energize, the proton pump in the first complex, fewer ATP molecules are made from the FADH2 electrons. The number of ATP molecules ultimately obtained is directly proportional to the number of protons pumped across the inner mitochondrial membrane.
Complex III
The third complex is composed of cytochrome b, another Fe-S protein, Rieske center (2Fe-2S center), and cytochrome c proteins; this complex is also called cytochrome oxidoreductase. Cytochrome proteins have a prosthetic heme group. The heme molecule is similar to the heme in hemoglobin, but it carries electrons, not oxygen. As a result, the iron ion at its core is reduced and oxidized as it passes the electrons, fluctuating between different oxidation states: Fe2+ (reduced) and Fe3+ (oxidized). The heme molecules in the cytochromes have slightly different characteristics due to the effects of the different proteins binding them, which makes each complex. Complex III pumps protons through the membrane and passes its electrons to cytochrome c for transport to the fourth complex of proteins and enzymes. Cytochrome c is the acceptor of electrons from Q; however, whereas Q carries pairs of electrons, cytochrome c can accept only one at a time.
Complex IV
The fourth complex is composed of cytochrome proteins c, a, and a3. This complex contains two heme groups (one in each of the cytochromes a and a3) and three copper ions (a pair of CuA and one CuB in cytochrome a3). The cytochromes hold an oxygen molecule very tightly between the iron and copper ions until the oxygen is completely reduced. The reduced oxygen then picks up two hydrogen ions from the surrounding medium to produce water (H2O). The removal of the hydrogen ions from the system also contributes to the ion gradient used in the process of chemiosmosis.
ATP Yield
The amount of energy (as ATP) gained from glucose catabolism varies across species and depends on other related cellular processes.
Learning Objectives
Describe the origins of variability in the amount of ATP that is produced per molecule of glucose consumed
Key Takeaways
Key Points
- While glucose catabolism always produces energy, the amount of energy (in terms of ATP equivalents) produced can vary, especially across different species.
- The number of hydrogen ions the electron transport chain complexes can pump through the membrane varies between species.
- NAD+ provides more ATP than FAD+ in the electron transport chain and can lead to variance in ATP production.
- The use of intermediates from glucose catabolism in other biosynthetic pathways, such as amino acid synthesis, can lower the yield of ATP.
Key Terms
- catabolism: Destructive metabolism, usually including the release of energy and breakdown of materials.
ATP Yield
In a eukaryotic cell, the process of cellular respiration can metabolize one molecule of glucose into 30 to 32 ATP. The process of glycolysis only produces two ATP, while all the rest are produced during the electron transport chain. Clearly, the electron transport chain is vastly more efficient, but it can only be carried out in the presence of oxygen.
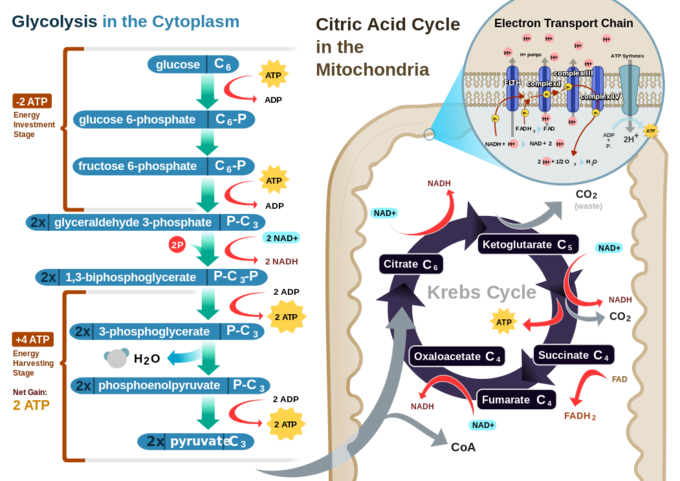
Cellular respiration in a eukaryotic cell: Glycolysis on the left portion of this illustration can be seen to yield 2 ATP molecules, while the Electron Transport Chain portion at the upper right will yield the remaining 30-32 ATP molecules under the presence of oxygen.
The number of ATP molecules generated via the catabolism of glucose can vary substantially. For example, the number of hydrogen ions the electron transport chain complexes can pump through the membrane varies between species. Another source of variance occurs during the shuttle of electrons across the membranes of the mitochondria. The NADH generated from glycolysis cannot easily enter mitochondria. Thus, electrons are picked up on the inside of mitochondria by either NAD+ or FAD+. These FAD+ molecules can transport fewer ions; consequently, fewer ATP molecules are generated when FAD+ acts as a carrier. NAD+ is used as the electron transporter in the liver, and FAD+ acts in the brain.
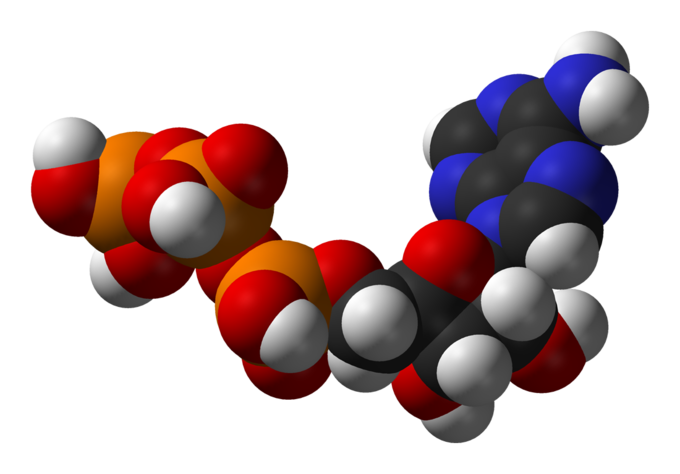
Adenosine triphosphate: ATP is the main source of energy in many living organisms.
Another factor that affects the yield of ATP molecules generated from glucose is the fact that intermediate compounds in these pathways are used for other purposes. Glucose catabolism connects with the pathways that build or break down all other biochemical compounds in cells, but the result is not always ideal. For example, sugars other than glucose are fed into the glycolytic pathway for energy extraction. Moreover, the five-carbon sugars that form nucleic acids are made from intermediates in glycolysis. Certain nonessential amino acids can be made from intermediates of both glycolysis and the citric acid cycle. Lipids, such as cholesterol and triglycerides, are also made from intermediates in these pathways, and both amino acids and triglycerides are broken down for energy through these pathways. Overall, in living systems, these pathways of glucose catabolism extract about 34 percent of the energy contained in glucose.
Control of Catabolic Pathways
Catabolic pathways are controlled by enzymes, proteins, electron carriers, and pumps that ensure that the remaining reactions can proceed.
Learning Objectives
Explain how catabolic pathways are controlled
Key Takeaways
Key Points
- Glycolysis, the citric acid cycle, and the electron transport chain are catabolic pathways that bring forth non-reversible reactions.
- Glycolysis control begins with hexokinase, which catalyzes the phosphorylation of glucose; its product is glucose-6- phosphate, which accumulates when phosphofructokinase is inhibited.
- The citric acid cycle is controlled through the enzymes that break down the reactions that make the first two molecules of NADH.
- The rate of electron transport through the electron transport chain is affected by the levels of ADP and ATP, whereas specific enzymes of the electron transport chain are unaffected by feedback inhibition.
Key Terms
- phosphofructokinase: any of a group of kinase enzymes that convert fructose phosphates to biphosphate
- glycolysis: the cellular metabolic pathway of the simple sugar glucose to yield pyruvic acid and ATP as an energy source
- kinase: any of a group of enzymes that transfers phosphate groups from high-energy donor molecules, such as ATP, to specific target molecules (substrates); the process is termed phosphorylation
Control of Catabolic Pathways
Enzymes, proteins, electron carriers, and pumps that play roles in glycolysis, the citric acid cycle, and the electron transport chain tend to catalyze non-reversible reactions. In other words, if the initial reaction takes place, the pathway is committed to proceeding with the remaining reactions. Whether a particular enzyme activity is released depends upon the energy needs of the cell (as reflected by the levels of ATP, ADP, and AMP).
Glycolysis
The control of glycolysis begins with the first enzyme in the pathway, hexokinase. This enzyme catalyzes the phosphorylation of glucose, which helps to prepare the compound for cleavage in a later step. The presence of the negatively-charged phosphate in the molecule also prevents the sugar from leaving the cell. When hexokinase is inhibited, glucose diffuses out of the cell and does not become a substrate for the respiration pathways in that tissue. The product of the hexokinase reaction is glucose-6-phosphate, which accumulates when a later enzyme, phosphofructokinase, is inhibited.
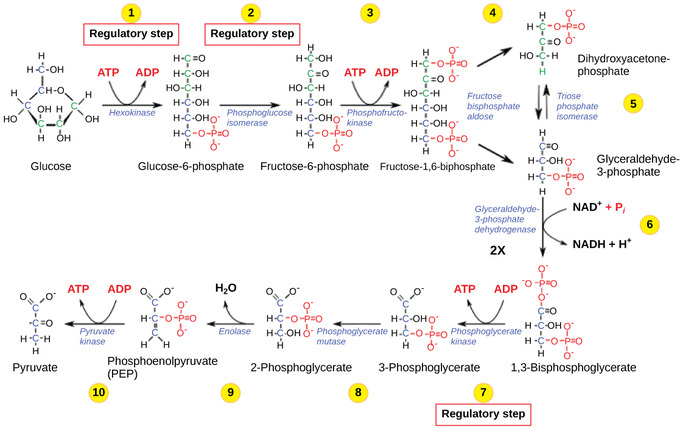
Glycolysis: The glycolysis pathway is primarily regulated at the three key enzymatic steps (1, 2, and 7) as indicated. Note that the first two steps that are regulated occur early in the pathway and involve hydrolysis of ATP.
Phosphofructokinase is the main enzyme controlled in glycolysis. High levels of ATP, citrate, or a lower, more acidic pH decrease the enzyme’s activity. An increase in citrate concentration can occur because of a blockage in the citric acid cycle. Fermentation, with its production of organic acids like lactic acid, frequently accounts for the increased acidity in a cell; however, the products of fermentation do not typically accumulate in cells.
The last step in glycolysis is catalyzed by pyruvate kinase. The pyruvate produced can proceed to be catabolized or converted into the amino acid alanine. If no more energy is needed and alanine is in adequate supply, the enzyme is inhibited. The enzyme’s activity is increased when fructose-1,6-bisphosphate levels increase. (Recall that fructose-1,6-bisphosphate is an intermediate in the first half of glycolysis. ) The regulation of pyruvate kinase involves phosphorylation, resulting in a less-active enzyme. Dephosphorylation by a phosphatase reactivates it. Pyruvate kinase is also regulated by ATP (a negative allosteric effect).
If more energy is needed, more pyruvate will be converted into acetyl CoA through the action of pyruvate dehydrogenase. If either acetyl groups or NADH accumulate, there is less need for the reaction and the rate decreases. Pyruvate dehydrogenase is also regulated by phosphorylation: a kinase phosphorylates it to form an inactive enzyme, and a phosphatase reactivates it. The kinase and the phosphatase are also regulated.
Citric Acid Cycle
The citric acid cycle is controlled through the enzymes that catalyze the reactions that make the first two molecules of NADH. These enzymes are isocitrate dehydrogenase and α-ketoglutarate dehydrogenase. When adequate ATP and NADH levels are available, the rates of these reactions decrease. When more ATP is needed, as reflected in rising ADP levels, the rate increases. α-Ketoglutarate dehydrogenase will also be affected by the levels of succinyl CoA, a subsequent intermediate in the cycle, causing a decrease in activity. A decrease in the rate of operation of the pathway at this point is not necessarily negative as the increased levels of the α-ketoglutarate not used by the citric acid cycle can be used by the cell for amino acid (glutamate) synthesis.
Citric Acid Cycle: Enzymes, isocitrate dehydrogenase and α-ketoglutarate dehydrogenase, catalyze the reactions that make the first two molecules of NADH in the citric acid cycle. Rates of the reaction decrease when sufficient ATP and NADH levels are reached.
Electron Transport Chain
Specific enzymes of the electron transport chain are unaffected by feedback inhibition, but the rate of electron transport through the pathway is affected by the levels of ADP and ATP. Greater ATP consumption by a cell is indicated by a buildup of ADP. As ATP usage decreases, the concentration of ADP decreases: ATP begins to build up in the cell. This change in the relative concentration of ADP to ATP triggers the cell to slow down the electron transport chain.
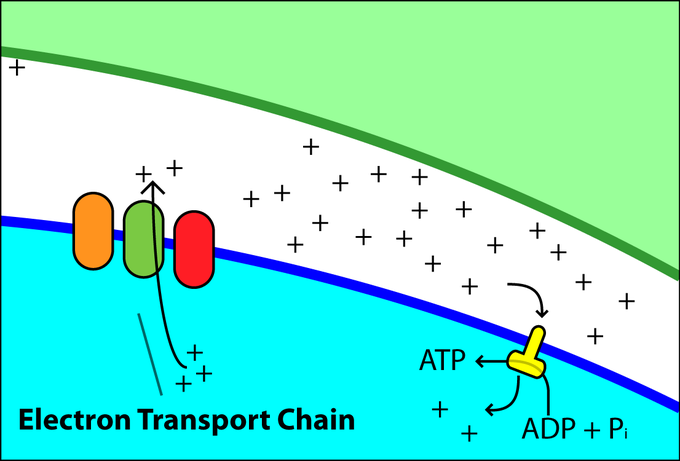
Electron Chain Transport: Levels of ADP and ATP affect the rate of electron transport through this type of chain transport.
Transforming Chemical Energy
Cellular respiration is the process of transforming chemical energy into forms usable by the cell or organism.
Learning Objectives
Discuss the importance of cellular respiration
Key Takeaways
Key Points
- Organisms ingest organic molecules like the carbohydrate glucose to obtain the energy needed for cellular functions.
- The energy in glucose can be extracted in a series of chemical reactions known as cellular respiration.
- Cellular respiration produces energy in the form of ATP, which is the universal energy currency for cells.
Key Terms
- aerobic respiration: the process of converting the biochemical energy in nutrients to ATP in the presence of oxygen
- adenosine triphosphate: a multifunctional nucleoside triphosphate used in cells as a coenzyme, often called the “molecular unit of energy currency” in intracellular energy transfer
- cellular respiration: the set of the metabolic reactions and processes that take place in the cells of organisms to convert biochemical energy from nutrients into adenosine triphosphate (ATP)
- catabolism: the breakdown of large molecules into smaller ones usually accompanied by the release of energy
Introduction: Cellular Respiration
An electrical energy plant converts energy from one form to another form that can be more easily used. For example, geothermal energy plants start with underground thermal energy (heat) and transform it into electrical energy that will be transported to homes and factories.
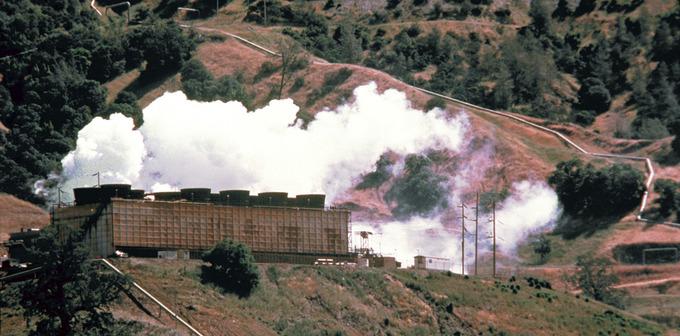
Energy Plant: This geothermal energy plant transforms thermal energy from deep in the ground into electrical energy, which can be easily used.
Like a generating plant, living organisms must take in energy from their environment and convert it into to a form their cells can use. Organisms ingest large molecules, like carbohydrates, proteins, and fats, and convert them into smaller molecules like carbon dioxide and water. This process is called cellular respiration, a form of catabolism, and makes energy available for the cell to use. The energy released by cellular respiration is temporarily captured by the formation of adenosine triphosphate (ATP) within the cell. ATP is the principle form of stored energy used for cellular functions and is frequently referred to as the energy currency of the cell.
The nutrients broken down through cellular respiration lose electrons throughout the process and are said to be oxidized. When oxygen is used to help drive the oxidation of nutrients the process is called aerobic respiration. Aerobic respiration is common among the eukaryotes, including humans, and takes place mostly within the mitochondria. Respiration occurs within the cytoplasm of prokaryotes. Several prokaryotes and a few eukaryotes use an inorganic molecule other than oxygen to drive the oxidation of their nutrients in a process called anaerobic respiration. Electron acceptors for anaerobic respiration include nitrate, sulfate, carbon dioxide, and several metal ions.
The energy released during cellular respiration is then used in other biological processes. These processes build larger molecules that are essential to an organism’s survival, such as amino acids, DNA, and proteins. Because they synthesize new molecules, these processes are examples of anabolism.