61 10.2 Global Geological Models of the Early 20th Century
The untimely death of Alfred Wegener didn’t solve any problems for those who opposed his ideas because they still had some inconvenient geological truths to deal with. One of those was explaining the distribution of terrestrial species across five continents that are currently separated by hundreds or thousands of kilometres of ocean water (Figure 10.1.2), and another was explaining the origin of extensive fold-belt mountains, such as the Appalachians, the Alps, the Himalayas, and the Canadian Rockies.
Before we go any further, it is important to know what was generally believed about global geology before plate tectonics. At the beginning of the 20th century, geologists had a good understanding of how most rocks were formed and understood their relative ages through interpretation of fossils, but there was considerable controversy regarding the origin of mountain chains, especially fold-belt mountains. At the end of the 19th century, one of the prevailing views on the origin of mountains was the theory of contractionism—the idea that since Earth is slowly cooling, it must also be shrinking. In this scenario, mountain ranges had formed like the wrinkles on a dried-up apple, and the oceans had submerged parts of former continents. While this theory helped to address the dilemma of the terrestrial fossils, it came with its own set of problems, one being that the amount of cooling couldn’t produce the necessary amount of shrinking, and the other being the principle of isostasy (which had already been around for several decades), which wouldn’t allow continents to sink. (See Section 9.4 for a review of the important principle of isostasy.)
Another widely held view was permanentism, in which it was believed that the continents and oceans have always been generally as they are today. This view incorporated a mechanism for creation of mountain chains known as the geosyncline theory. A geosyncline is a thick deposit of sediments and sedimentary rocks, typically situated along the edge of a continent (Figure 10.2.1).
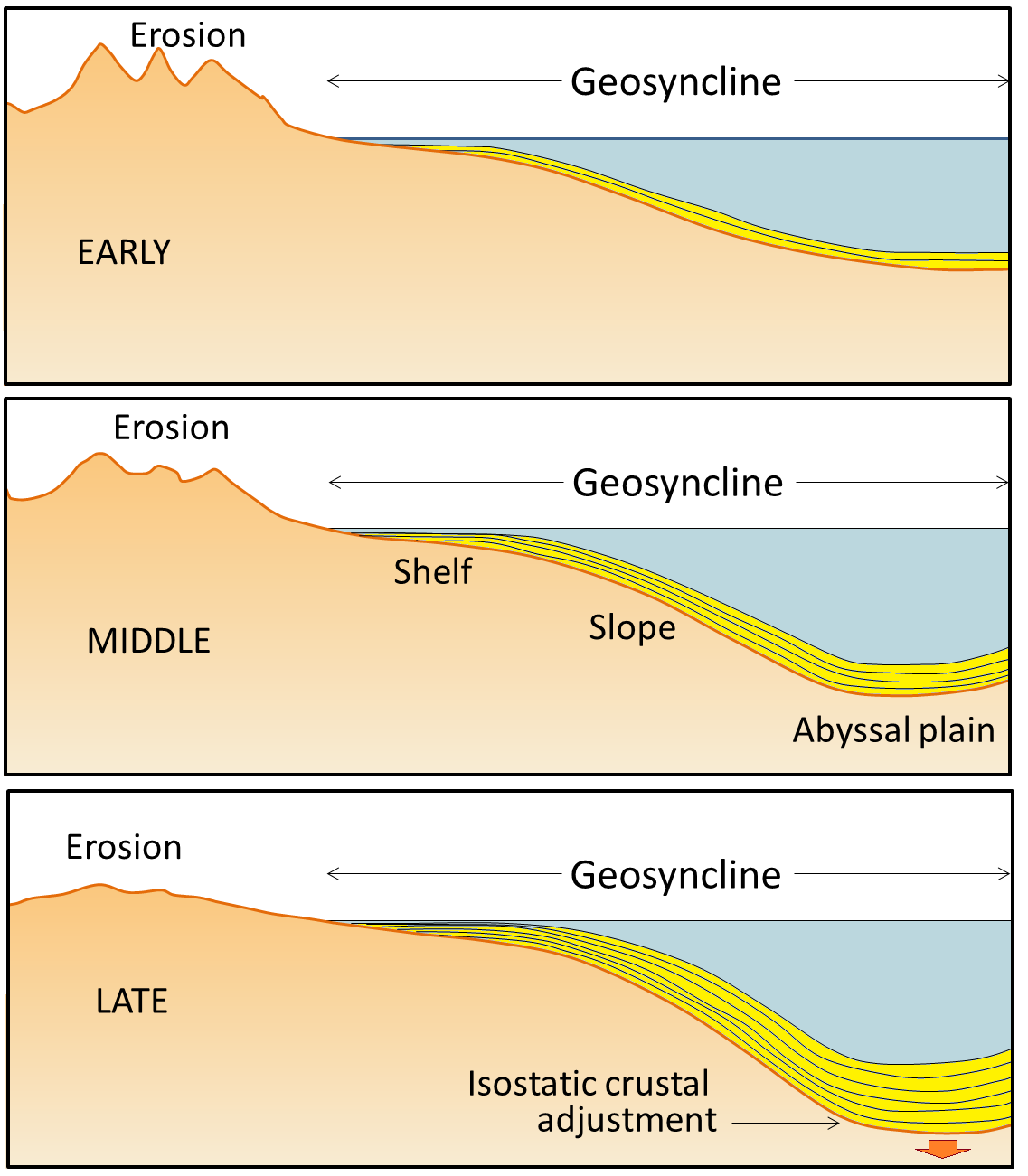
The idea of geosynclines developing into fold-belt mountains originated in the middle of the 19th century, proposed first by James Hall and later elaborated by Dwight Dana, both of whom worked extensively in the Appalachian Mountains of the eastern United States. The process of converting a geosyncline into a mountain belt was never really adequately explained, although it was widely believed that mountain belts formed when geosynclines were compressed by forces pushing from either side. The problem is that, without the lateral forces related to plate tectonics, no one was able to adequately describe what would do the pushing. The sediments that accumulate within a geosyncline are derived from erosion of the adjacent continent. Geosynclinal sediments—which eventually turn into sedimentary rocks—may be many thousands of metres thick. As they accumulate, they push down the pre-existing crustal rocks. Extensive geosynclinal deposits exist around much of the coastline of most of the continents; there is a large geosyncline along the eastern edge of North America.
Proponents of the geosyncline theory of mountain formation—and there were many well into the 1960s—also had the problem of explaining the intercontinental terrestrial fossil matchups. The simple explanation was that there were “land bridges” across the Atlantic along which animals and plants could migrate back and forth. One proponent of this idea was the American naturalist Ernest Ingersoll. Referring to evidence of past climate changes, Ingersoll contributed the following to the Encyclopedia Americana in 1920: “The most interesting feature of these changes, however, is that by which, now and again, the Old World was connected with the New by necks or spaces of land, known as “land-bridges”; especially as these permitted an interchange of plants and animals, giving to us many new ones from the other side of the ocean, including, finally, man himself.”[1]
There are serious problems with the land-bridge theory. One is that it is completely inconsistent with isostasy, and another is that there is no evidence of the remnants of the land bridges. The Atlantic Ocean is several thousand metres deep over wide areas, and so the underwater slopes leading up to a land bridge would have to have been at least tens of kilometres wide in most places, and many times that in others. A land bridge of that size would certainly have left some trace.
Exercise 10.1 Fitting the continents together

The main continents around the Atlantic Ocean are depicted here in the shapes that they might have had during the Mesozoic, including the extents of their continental shelves. Cut these shapes out and see how well you can fit them together in the positions that these areas occupied within Pangea. You can refer to a map of Pangea to help you make the fit.
See Appendix 3 for Exercise 10.1 answers.
Media Attributions
- Figures 10.2.1, 10.2.2: © Steven Earle. CC BY.
- http://en.wikisource.org/wiki/The_Encyclopedia_Americana_(1920)/Land-Bridges_Across_the_Oceans ↵
There are numerous types of volcanoes or volcanic sources; some of the more common ones are summarized in Table 4.1.
[Skip Table] | ||||
Type | Tectonic Setting | Size and Shape | Magma and Eruption Characteristics | Example |
---|---|---|---|---|
Cinder cone | Various; some form on the flanks of larger volcanoes | Small (10s to 100s of metres) and steep (Greater than 20°) | Most are mafic and form from the gas-rich early stages of a shield- or rift-associated eruption | Eve Cone, northern B.C. |
Composite volcano | Almost all are at subduction zones | Medium size (1000s of metres high and up to 20 km across) and moderate steepness (10° to 30°) | Magma composition varies from felsic to mafic, and from explosive to effusive | Mount St. Helens |
Shield volcano | Most are at mantle plumes; some are on spreading ridges | Large (up to several 1,000 metres high and up to 200 kilometres across), not steep (typically 2° to 10°) | Magma is almost always mafic, and eruptions are typically effusive, although cinder cones are common on the flanks of shield volcanoes | Kilauea, Hawaii |
Large igneous provinces | Associated with “super” mantle plumes | Enormous (up to millions of square kiometres) and 100s of metres thick | Magma is always mafic and individual flows can be 10s of metres thick | Columbia River basalts |
Sea-floor volcanism | Generally associated with spreading ridges but also with mantle plumes | Large areas of the sea floor associated with spreading ridges | Pillows form at typical eruption rates; lava flows develop if the rare of flow is faster | Juan de Fuca ridge |
Kimberlite | Upper-mantle sourced | The remnants are typically 10s to 100s of metres across | Most appear to have had explosive eruptions forming cinder cones; the youngest one is dated at about 10 ka, and all others are at least 30 Ma | Lac de Gras Kimberlite Field, N.W.T. |
The sizes and shapes of typical shield, composite, and cinder-cone volcanoes are compared in Figure 4.3.1, although, to be fair, Mauna Loa is the largest shield volcano on Earth; all others are smaller. Mauna Loa rises from the surrounding flat sea floor, and its diameter is in the order of 200 km. Its elevation is 4,169 m above sea level. Mount St. Helens, a composite volcano of average size, rises above the surrounding hills of the Cascade Range. Its diameter is about 6 km, and its height is 2,550 m above sea level. Cinder cones are much smaller. On this drawing, even a large cinder cone is just a dot.

Cinder Cones
Cinder cones, like Eve Cone in northern B.C. (Figure 4.3.2), are typically only a few hundred metres in diameter, and few are more than 200 m high. Most are made up of fragments of vesicular mafic rock (scoria) that were expelled as the magma boiled when it approached the surface, creating fire fountains. In many cases, these later became the sites of effusive lava flows when the gases were depleted. Most cinder cones are monogenetic, meaning that they formed during a single eruptive phase that might have lasted weeks or months. Because cinder cones are made up almost exclusively of loose fragments, they have very little strength. They can be easily, and relatively quickly, eroded away.
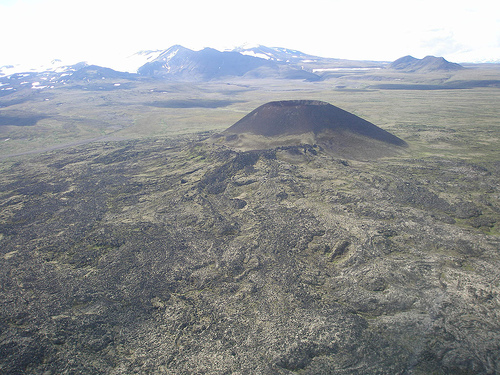
Composite Volcanoes
Composite volcanoes, like Mount St. Helens in Washington State (Figure 4.3.3), are almost all associated with subduction at convergent plate boundaries—either ocean-continent or ocean-ocean boundaries (Figure 4.1.2b). They can extend up to several thousand metres from the surrounding terrain, and, with slopes ranging up to 30˚ They can be up to about 20 km across. At many such volcanoes, magma is stored in a magma chamber in the upper part of the crust. For example, at Mount St. Helens, there is evidence of a magma chamber that is approximately 1 kilometre wide and extends from about 6 km to 14 km below the surface (Figure 4.3.4). Systematic variations in the composition of volcanism over the past several thousand years at Mount St. Helens imply that the magma chamber is zoned, from more felsic at the top to more mafic at the bottom.

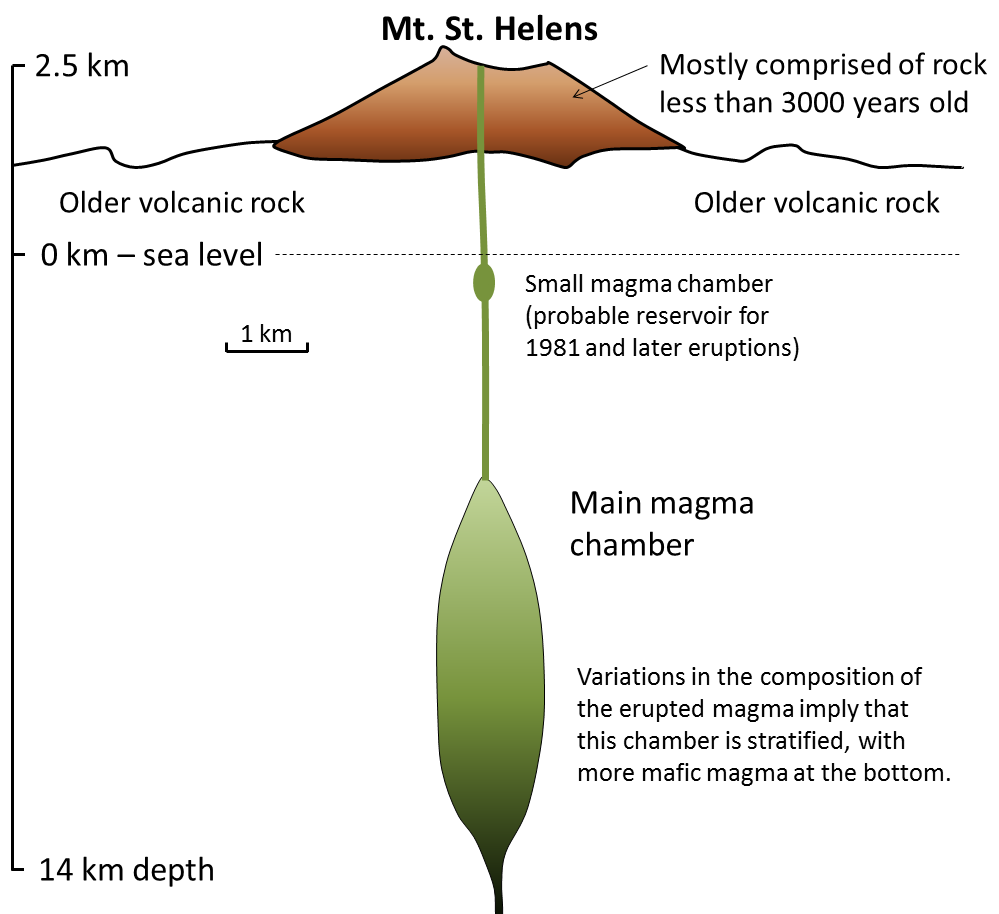
Mafic eruptions (and some intermediate eruptions), on the other hand, produce lava flows; the one shown in Figure 4.3.5b is thick enough (about 10 m in total) to have cooled in a columnar jointing pattern (Figure 4.3.7). Lava flows both flatten the profile of the volcano (because the lava typically flows farther than pyroclastic debris falls) and protect the fragmental deposits from erosion. Even so, composite volcanoes tend to erode quickly. Patrick Pringle, a volcanologist with the Washington State Department of Natural Resources, describes Mount St. Helens as a “pile of junk.” The rock that makes up Mount St. Helens ranges in composition from rhyolite (Figure 4.3.5a) to basalt (Figure 4.3.5b); this implies that the types of past eruptions have varied widely in character. As already noted, felsic magma doesn’t flow easily and doesn’t allow gases to escape easily. Under these circumstances, pressure builds up until a conduit opens, and then an explosive eruption results from the gas-rich upper part of the magma chamber, producing pyroclastic debris, as shown on Figure 4.3.5a. This type of eruption can also lead to rapid melting of ice and snow on a volcano, which typically triggers large mudflows known as lahars (Figure 4.3.5a). Hot, fast-moving pyroclastic flows and lahars are the two main causes of casualties in volcanic eruptions. Pyroclastic flows killed approximately 30,000 people during the 1902 eruption of Mount. Pelée on the Caribbean island of Martinique. Most were incinerated in their homes. In 1985 a massive lahar, triggered by the eruption of Nevado del Ruiz, killed 23,000 people in the Colombian town of Armero, about 50 km from the volcano.
In a geological context, composite volcanoes tend to form relatively quickly and do not last very long. Mount St. Helens, for example, is made up of rock that is all younger than 40,000 years; most of it is younger than 3,000 years. If its volcanic activity ceases, it might erode away within a few tens of thousands of years. This is largely because of the presence of pyroclastic eruptive material, which is not strong.
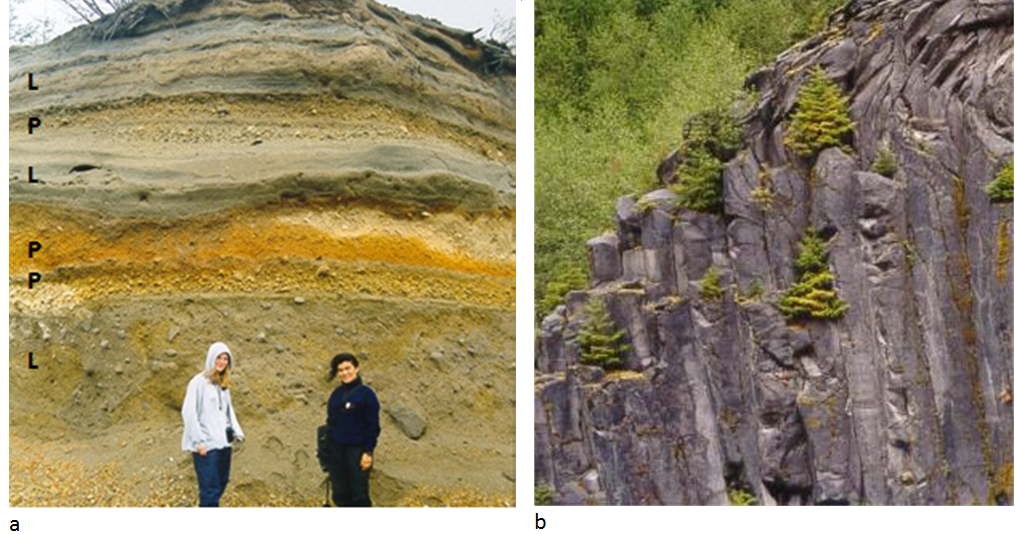
Exercise 4.3 Volcanoes and Subduction
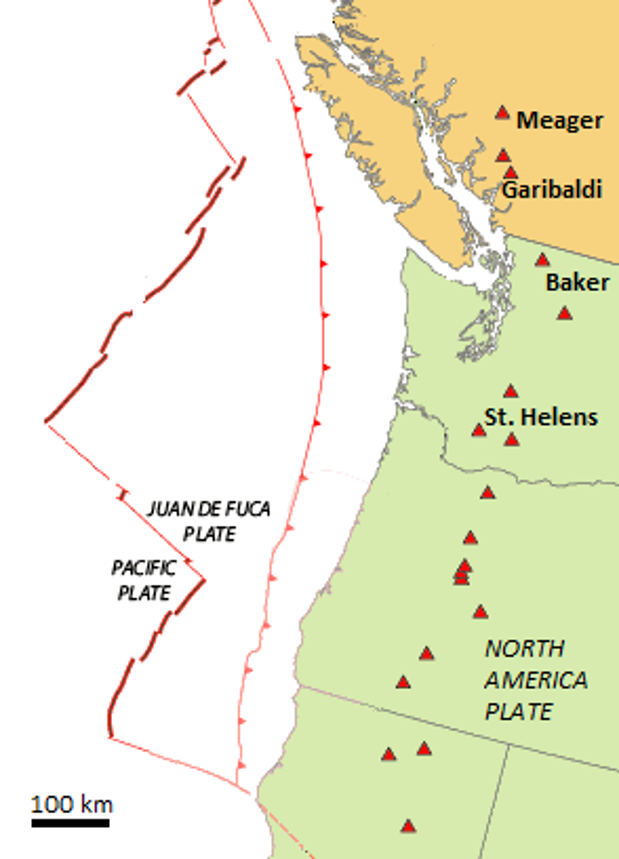
The map shown here illustrates the interactions between the North America, Juan de Fuca, and Pacific Plates off the west coast of Canada and the United States. The Juan de Fuca Plate is forming along the Juan de Fuca ridge, and is then subducted beneath the North America Plate along the red line with teeth on it (“Subduction boundary”).
- Using the scale bar in the lower left of the map, estimate the average distance between the subduction boundary and the Cascadia composite volcanoes.
- If the subducting Juan de Fuca Plate descends 40 km for every 100 km that it moves inland, what is its likely depth in the area where volcanoes are forming?
See Appendix 3 for Exercise 4.3 answers.

Shield Volcanoes
Most shield volcanoes are associated with mantle plumes, although some form at divergent boundaries, either on land or on the sea floor. Because of their non-viscous mafic magma they tend to have relatively gentle slopes (2 to 10˚) and the larger ones can be over 100 km in diameter. The best-known shield volcanoes are those that make up the Hawaiian Islands, and of these, the only active ones are on the big island of Hawaii. Mauna Loa, the world’s largest volcano and the world’s largest mountain (by volume) last erupted in 1984. Kilauea, arguably the world’s most active volcano, has been erupting, virtually without interruption, since 1983. Loihi is an underwater volcano on the southeastern side of Hawaii. It is last known to have erupted in 1996, but may have erupted since then without being detected.
All of the Hawaiian volcanoes are related to the mantle plume that currently lies beneath Mauna Loa, Kilauea, and Loihi (Figure 4.3.8). In this area, the Pacific Plate is moving northwest at a rate of about 7 centimetres (cm) per year. This means that the earlier formed — and now extinct — volcanoes have now moved well away from the mantle plume. As shown on Figure 4.3.8, there is evidence of crustal magma chambers beneath all three active Hawaiian volcanoes. At Kilauea, the magma chamber appears to be several kilometres in diameter, and is situated between 8 km and 11 km below surface.[1]
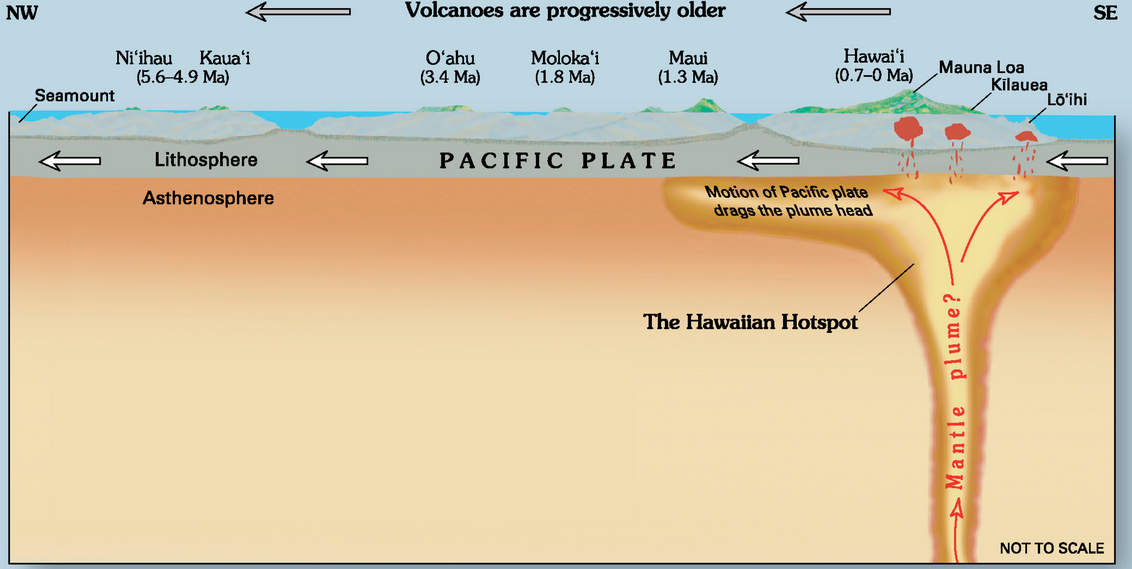
Although it is not a prominent mountain (Figure 4.3.2), Kilauea volcano has a large caldera in its summit area (Figure 4.3.9). A caldera is a volcanic crater that is more than 2 km in diameter; this one is 4 km long and 3 km wide. It contains a smaller feature called Halema’uma’u crater, which has a total depth of over 200 m below the surrounding area. Most volcanic craters and calderas are formed above magma chambers, and the level of the crater floor is influenced by the amount of pressure exerted by the magma body. During historical times, the floors of both Kilauea caldera and Halema’uma’u crater have moved up during expansion of the magma chamber and down during deflation of the chamber.
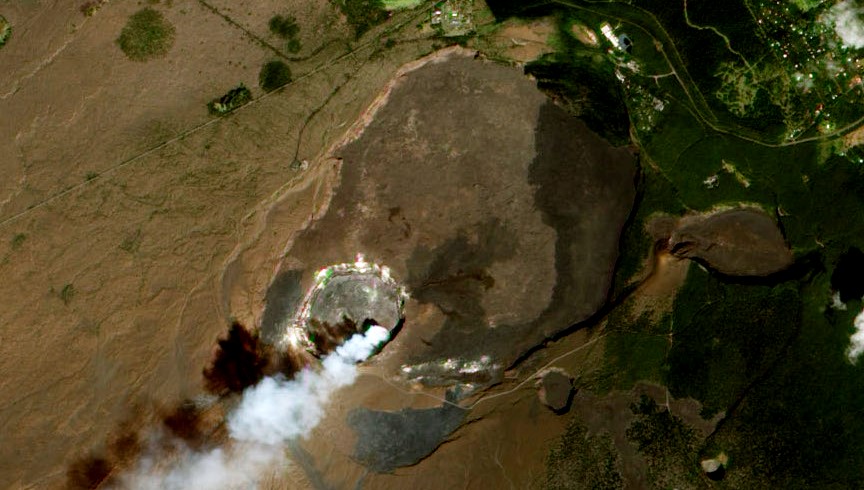
One of the conspicuous features of Kilauea caldera is rising water vapour (the white cloud in Figure 4.3.9) and a strong smell of sulphur (Figure 4.3.10). As is typical in magmatic regions, water is the main volatile component, followed by carbon dioxide and sulphur dioxide. These, and some minor gases, originate from the magma chamber at depth and rise up through cracks in the overlying rock. This degassing of the magma is critical to the style of eruption at Kilauea, which, for most of the past 35 years, has been effusive, not explosive.
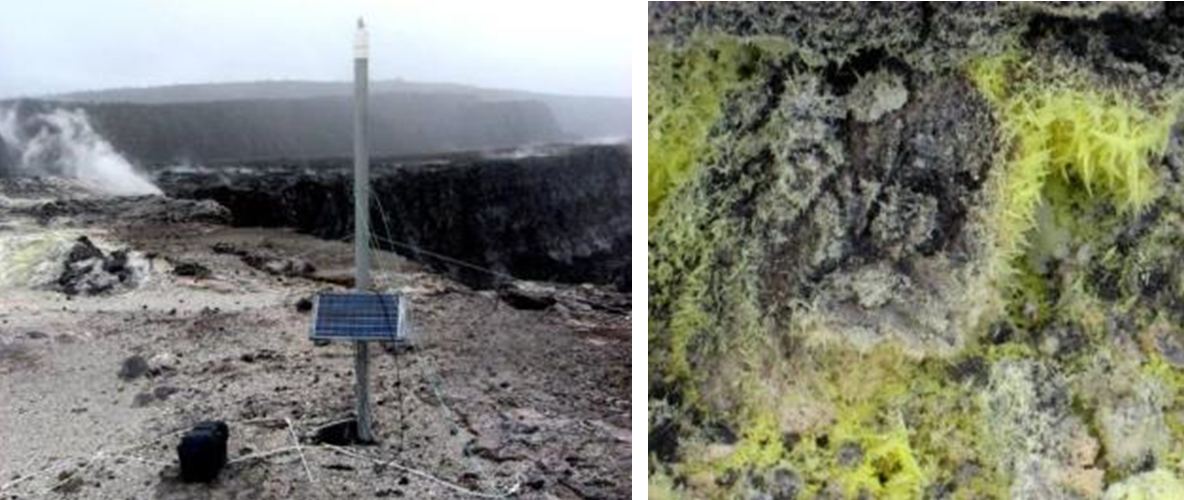
The Kilauea eruption that began in 1983 started with the formation of a cinder cone at Pu’u ’O’o, approximately 15 km east of the caldera (Figure 4.3.11). The magma feeding this eruption flowed along a major conduit system known as the East Rift, which extends for about 20 km from the caldera, first southeast and then east. Lava fountaining and construction of the Pu’u ’O’o cinder cone (Figure 4.3.12a) continued until 1986 at which time the flow became effusive. From 1986 to 2014, lava flowed from a gap in the southern flank of Pu’u ’O’o down the slope of Kilauea through a lava tube (Figure 4.3.12d), emerging at or near the ocean. During 2014 and 2015, the lava flowed northeast toward the community of Pahoa (see Exercise 4.4). In May of 2018 a new eruption started another 15 km east of the 2014/15 flow in the area known as Leilani Estates. The Lower East Rift Flow was active for 6 months. During that time, 35 km2 of existing land was covered in lava and 3.5 km2 of new land was created (Figure 4.3.11), about 48 km of road were covered in lava and 716 dwellings were destroyed (see USGS Overview of Kilauea Volcanoe's 2018 eruption[PDF]). Volcanic activity on the East Rift ceased in August 2018, and there has been no activity on Kilauea since then. This appears to mark the end of the eruption cycle that lasted—with only a few short interruptions—for 35 years. Kilauea will almost certainly erupt again within years or decades.
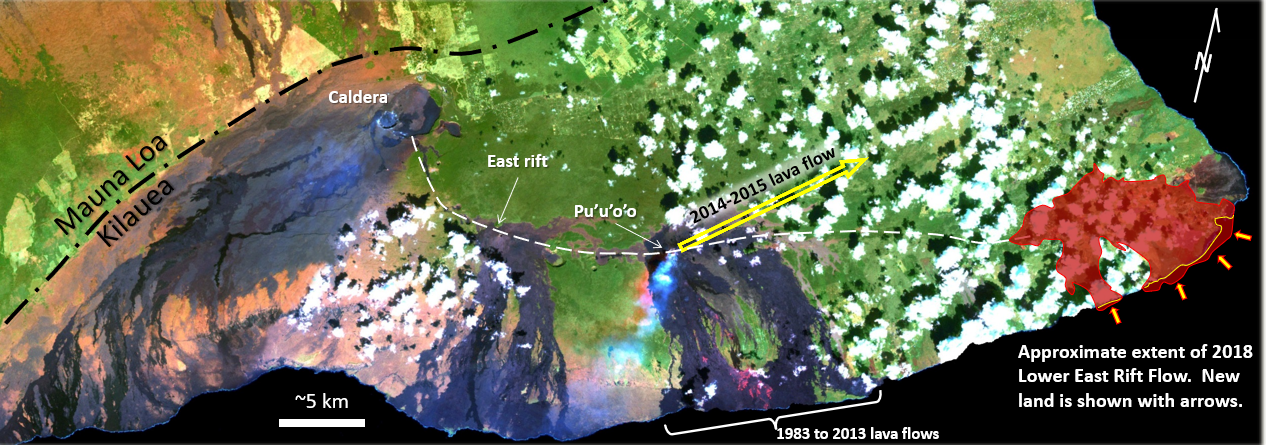
The two main types of textures created during effusive subaerial eruptions are pahoehoe and aa. Pahoehoe, ropy lava that forms as non-viscous lava, flows gently, forming a skin that gels and then wrinkles because of ongoing flow of the lava below the surface (Figure 4.3.12b, and "lava flow video"). Aa, or blocky lava, forms when magma is forced to flow faster than it is able to (down a slope for example) (Figure 4.3.12c). Tephra (lava fragments) is produced during explosive eruptions, and accumulates in the vicinity of cinder cones.
Figure 4.3.12d is a view into an active lava tube on the southern edge of Kilauea. The red glow is from a stream of very hot lava (~1200°C) that has flowed underground for most of the 8 km from the Pu’u ’O’o vent. Lava tubes form naturally and readily on both shield and composite volcanoes because flowing mafic lava preferentially cools near its margins, forming solid lava levées that eventually close over the top of the flow. The magma within a lava tube is not exposed to the air, so it remains hot and fluid and can flow for tens of km, thus contributing to the large size and low slopes of shield volcanoes. The Hawaiian volcanoes are riddled with thousands of old lava tubes, some as long as 50 km.

Kilauea started forming at approximately 300 ka, while neighbouring Mauna Loa dates back to 700 ka and nearby Mauna Kea ito around 1 Ma. If volcanism continues above the Hawaii mantle plume in the same manner that it has since 85 Ma, it is likely that Kilauea will continue to erupt for at least another 500,000 years. By that time, its neighbour, Loihi, will have emerged from the sea floor, and its other neighbours, Mauna Loa and Mauna Kea, will have become significantly eroded, like their cousins, the islands to the northwest (Figure 4.3.8).
Exercise 4.4 Kilauea's 2014 lava flow
The U.S. Geological Survey Hawaii Volcano Observatory (HVO) map shown here, dated January 29, 2015, shows the outline of lava that started flowing northeast from Pu’u ’O’o on June 27, 2014 (the “June 27th Lava flow,” a.k.a. the “East Rift Lava Flow”). The flow reached the nearest settlement, Pahoa, on October 29, 124 days later. After damaging some infrastructure west of Pahoa, the flow stopped advancing. A new outbreak occurred November 1, branching out to the north from the main flow.
What is the average rate of advance of the flow front from June 27 to October 29, 2014, in metres per day and metres per hour?
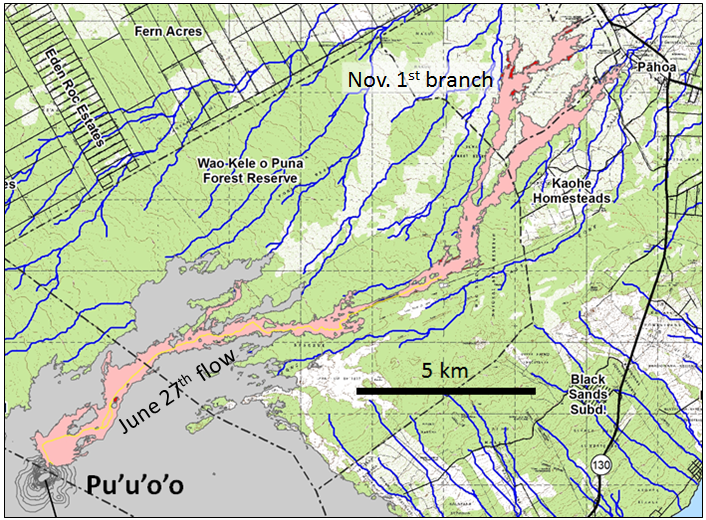
See Appendix 3 for Exercise 4.4 Answers.
Large Igneous Provinces
While the Hawaii mantle plume has produced a relatively low volume of magma for a very long time (~85 Ma), other mantle plumes are less consistent, and some generate massive volumes of magma over relatively short time periods. Although their origin is still controversial, it is thought that the volcanism leading to large igneous provinces (LIP) is related to very high volume but relatively short duration bursts of magma from mantle plumes. An example of an LIP is the Columbia River Basalt Group (CRGB), which extends across Washington, Oregon, and Idaho (Figure 4.3.14). This volcanism, which covered an area of about 160,000 square kilometres (km2) with basaltic rock up to several hundred metres thick, took place between 17 and 14 Ma.
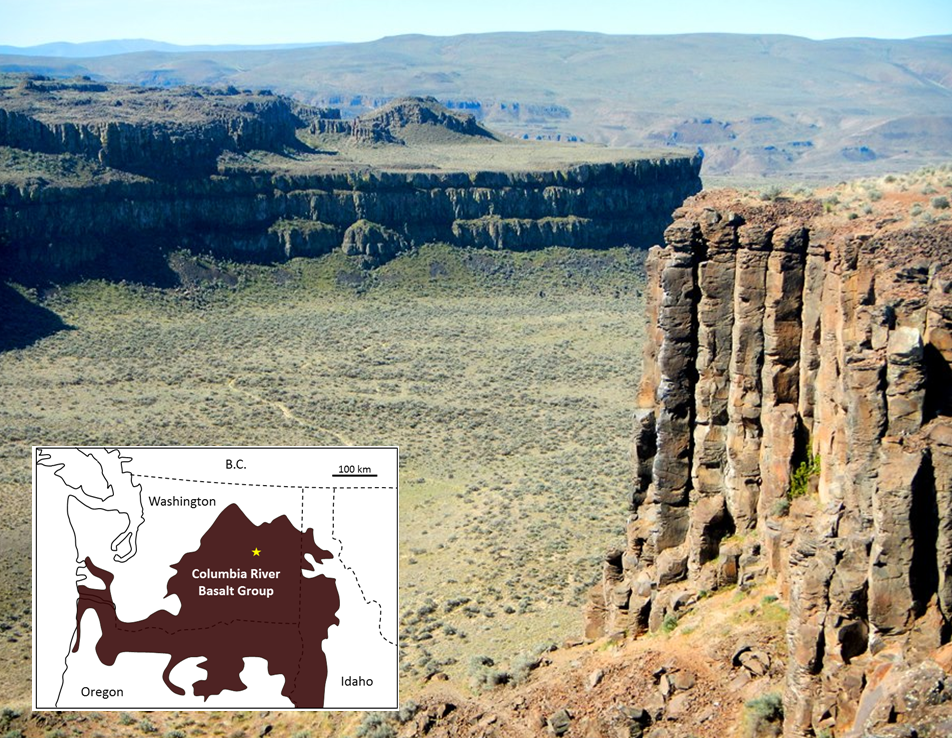
Most other LIP eruptions are much bigger. The Siberian Traps (also basalt), which erupted at the end of the Permian period at 250 Ma, are estimated to have produced approximately 40 times as much lava as the CRBG.
The mantle plume that is assumed to be responsible for the CRBG is now situated beneath the Yellowstone area, where it leads to felsic volcanism. Over the past 2 Ma three very large explosive eruptions at Yellowstone have yielded approximately 900 cubic kilometres (km3) of felsic magma, about 900 times the volume of the 1980 eruption of Mount St. Helens, but only 5% of the volume of mafic magma in the CRBG.
Sea-Floor Volcanism
Some LIP eruptions occur on the sea floor, the largest known being the one that created the Ontong Java plateau in the western Pacific Ocean at around 122 Ma. But most sea-floor volcanism originates at divergent boundaries and involves relatively low-volume eruptions. Under these conditions, hot lava that oozes out into the cold seawater quickly cools on the outside and then behaves a little like toothpaste. The resulting blobs of lava are known as pillows, and they tend to form piles around a sea-floor lava vent (Figure 4.3.15). In terms of area, there is very likely more pillow basalt on the sea floor than any other type of rock on Earth.
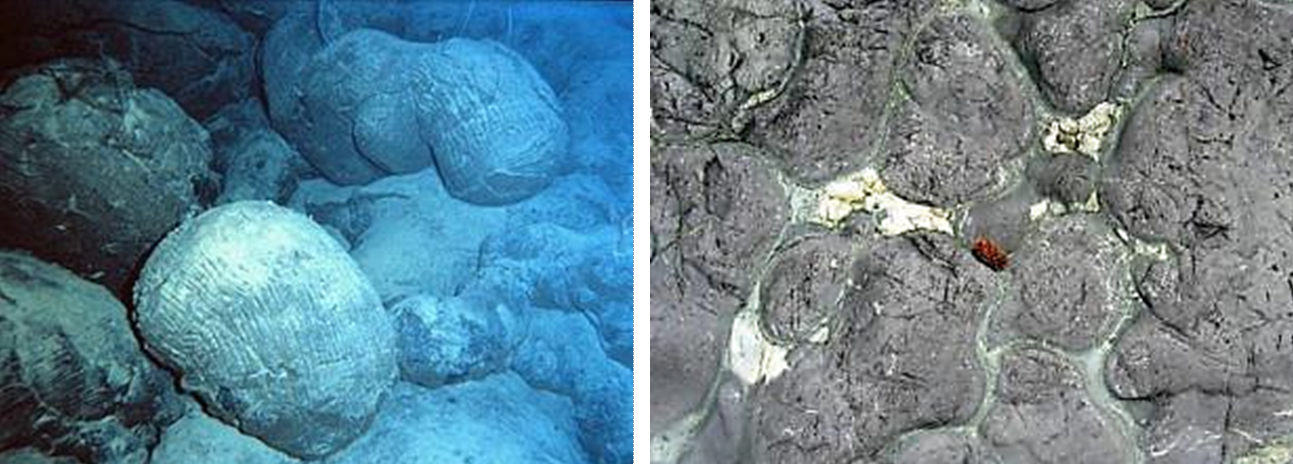
Kimberlites
While all of the volcanism discussed so far is thought to originate from partial melting in the upper mantle or within the crust, there is a special class of volcanoes called kimberlites that have their origins much deeper in the mantle, at depths of 150 km to 450 km. During a kimberlite eruption, material from this depth may make its way to surface quickly (hours to days) with little interaction with the surrounding rocks. As a result, kimberlite eruptive material is representative of mantle compositions: it is ultramafic.
Kimberlite eruptions that originate at depths greater than 200 km, within areas beneath old thick crust (shields), traverse the region of stability of diamond in the mantle, and in some cases, bring diamond-bearing material to the surface. All of the diamond deposits on Earth are assumed to have formed in this way; an example is the rich Ekati Mine in the Northwest Territories (Figure 4.3.16).
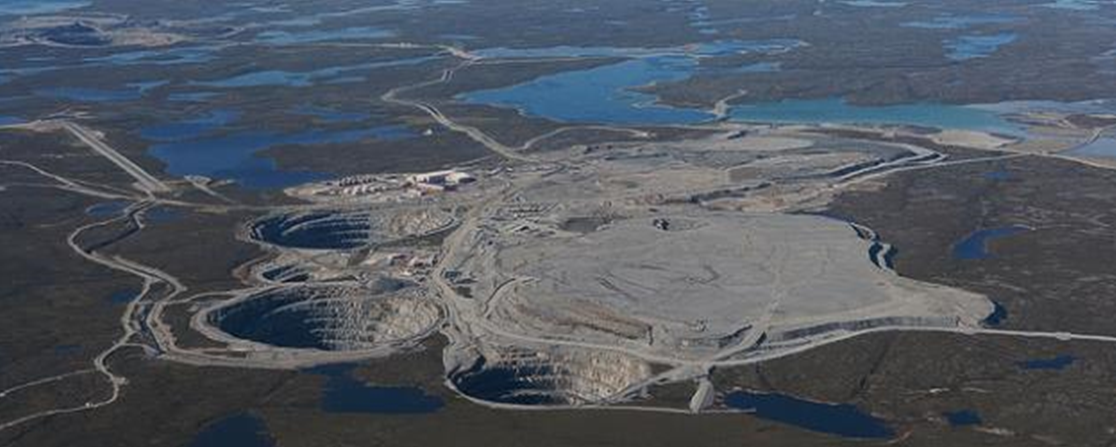
The kimberlites at Ekati erupted between 45 and 60 Ma. Many kimberlites are older, some much older. There have been no kimberlite eruptions in historic times. The youngest known kimberlites are in the Igwisi Hills in Tanzania and are only about 10,000 years old. The next youngest known are dated to about 30 Ma.
How frequently do volcanoes erupt?
The Smithsonian Institution maintains a comprehensive catalogue of the world's volcanoes, with information and eruptive history for nearly 2700 volcanic sites. If you spend some time looking around that site you'll discover the frequency of eruptions at different volcanoes is enormously variable, although we can make some generalizations. Focusing just on shield volcanoes and composite volcanoes some of the data are as follows:
Composite volcanoes | Shield volcanoes |
---|---|
Avachinsky (Russia): 5 eruptions over the past 7000 years | Fernandina (Galapagos): 31 eruptions over the past 1000 years |
Pinatubo (Philippines): 4 eruptions over the past 9000 years | Kilauea (Hawaii): 62 eruptions over the past 250 years |
Adams (Oregon, USA): 6 eruptions over the past 7000 years | Nyamuragira (Congo): 48 eruptions over the past 154 years |
Based only on these numbers it is evident that, in general, shield volcanoes are much more active than composite volcanoes, but there are many exceptions to this trend. Some composite volcanoes are nearly as active as the shield volcanoes listed here, and some shield volcanoes that are still considered to be "active" are almost as inactive as the composite volcanoes listed here.
Image Descriptions
Figure 4.3.4 image description: Mount St. Helens rises over 2.5 kilometres above sea lever and consists mostly of rock less than 3,000 years old. Underneath the mountain is older volcanic rock. Just below sea level is a small magma chamber, which is a probable reservoir for 1981 and later eruptions. Down 5 to 14 kilometres below sea level is the main magma chamber. Variations in the composition of the erupted magma imply this chamber is stratified, with more magma at the bottom. [Return to Figure 4.3.4]
Figure 4.3.5 image description: Image (A) shows a cliff wall with grey/brown and orange horizontal layers. The sides look soft like they would be easily worn away. The grey/brown layers are lahar deposits and the orange layers are felsic pyroclastic deposits. Image (B) shows a columnar basalt lava flow which looks like a rocky, stone cliff with vertical layers. [Return to Figure 4.3.5]
Figure 4.3.13 image description: The U.S. Geological Survey Hawaii Volcano Observatory (HVO) map, dated January 29, 2015, shows the outline of lava that started flowing northeast from Pu’u ’O’o on June 27, 2004 (the “June 27th Lava flow,” a.k.a. the “East Rift Lava Flow”). The flow reached the nearest settlement, Pahoa, on October 29, after covering a distance of 20 km in 124 days. After damaging some infrastructure west of Pahoa, the flow stopped advancing. A new outbreak occurred November 1, branching out to the north from the main flow about 6 km southwest of Pahoa. [Return to Figure 4.3.13]
Figure 4.3.14 image description: The Columbia River Basalt Group covers most of south eastern Washington state and stretches along the borders between Washington, Idaho, and Oregon. The columnar basalts shown in the photo are in in eastern Washington. They rise up out of a flat valley as tall cliffs. [Return to Figure 4.3.14]
Media Attributions
- Figure 4.3.1: © Steven Earle. CC BY.
- Figure 4.3.2: Eve Cone © nass5518. CC BY.
- Figure 4.3.3: © Steven Earle. CC BY.
- Figure 4.3.4: Original image © Pringle, 1993. Modified by Steve Earle.
- Figure 4.3.5: © Steven Earle. CC BY.
- Figure 4.3.6: © Steven Earle. CC BY.
- Figure 4.3.7: © Steven Earle. CC BY.
- Figure 4.3.8: "Hawaii hotspot cross-sectional diagram" by USGS. Public domain.
- Figure 4.3.9: "Kilauea ali 2012 01 28" by NASA. Public domain.
- Figure 4.3.10: © Steven Earle. CC BY.
- Figure 4.3.11: Island of Hawai'i - Landsat mosaic by NOAA. Public domain. Modified by Steven Earle.
- Figure 4.3.12: © Steven Earle. CC BY.
- Figure 4.3.13: Image from USGS. Public domain.
- Figure 4.3.14: © Steven Earle. CC BY.
- Figure 4.3.15 (Left): Pillow Basalt Crop by NOAA. Public domain.
- Figure 4.3.15 (Right): © Steven Earle. CC BY.
- Figure 4.3.16: "Ekati mine" © Jason Pineau. CC BY-SA.
There are two classes of volcanic hazards, direct and indirect. Direct hazards are forces that directly kill or injure people, or destroy property or wildlife habitat. Indirect hazards are volcanism-induced environmental changes that lead to distress, famine, or habitat degradation. It is estimated that indirect effects of volcanism have accounted for approximately 8 million deaths during historical times, while direct effects have accounted for fewer than 200,000, or 2.5% of the total. Some of the more important types of volcanic hazards are summarized in Table 4.3.
[Skip Table] | ||
Type | Description | Risk |
---|---|---|
Tephra emissions | Small particles of volcanic rock emitted into the atmosphere |
|
Gas emissions | The emission of gases before, during, and after an eruption |
|
Pyroclastic density current | A very hot (several 100°C) mixture of gases and volcanic fragments (tephra) that flows rapidly (up to 100s of kilometres per hour (km/h)) down the side of a volcano | Extreme hazard — destroys anything in the way |
Pyroclastic fall | Vertical fall of tephra in the area surrounding an eruption |
|
Lahar | A flow of mud and debris down a channel leading away from a volcano, triggered either by an eruption or a severe rain event | Severe risk of destruction for anything within the channel—lahar mud flows can move at 10s of km/h |
Sector collapse/ debris avalanche | The failure of part of a volcano, either due to an eruption or for some other reason, leading to the failure of a large portion of the volcano | Severe risk of destruction for anything in the path of the debris avalanche |
Lava flow | The flow of lava away from a volcanic vent | People and infrastructure at risk, but lava flows tend to be slow (less than km/h) and are relatively easy to avoid |
Volcanic Gas and Tephra Emissions
Large volumes of tephra (rock fragments, mostly pumice) and gases are emitted during major plinian eruptions (large explosive eruptions with hot gas and tephra columns extending into the stratosphere) at composite volcanoes, and a large volume of gas is released during some very high-volume effusive eruptions. One of the major effects is cooling of the climate by 1° to 2°C for several months to a few years because the dust particles and tiny droplets and particles of sulphur compounds block the sun. The last significant event of this type was in 1991 and 1992 following the large eruption of Mount Pinatubo in the Philippines. A temperature decrease of 1° to 2°C may not seem like very much, but that is the global average amount of cooling, and cooling was much more severe in some regions and at some times.
Over an eight-month period in 1783 and 1784, a massive effusive eruption took place at the Laki volcano in Iceland. Although there was relatively little volcanic ash involved, a massive amount of sulphur dioxide was released into the atmosphere, along with a significant volume of hydrofluoric acid (HF). The sulphate aerosols that formed in the atmosphere led to dramatic cooling in the northern hemisphere. There were serious crop failures in Europe and North America, and a total of 6 million people are estimated to have died from famine and respiratory complications. In Iceland, poisoning from the HF resulted in the death of 80% of sheep, 50% of cattle, and the ensuing famine, along with HF poisoning, resulted in more than 10,000 human deaths—about 25% of the population.
Volcanic ash can also have serious implications for aircraft because it can destroy jet engines. For example, over 5 million airline passengers had their travel disrupted by the 2010 Eyjafjallajökull volcanic eruption in Iceland.
Pyroclastic Density Currents
In a typical explosive eruption at a composite volcano, the tephra and gases are ejected with explosive force and are hot enough to be forced high up into the atmosphere. As the eruption proceeds, and the amount of gas in the rising magma starts to decrease, parts will become heavier than air, and they can then flow downward along the flanks of the volcano (Figure 4.4.1). As they descend, they cool more and flow faster, reaching speeds up to several hundred kilometres per hour. A pyroclastic density current (PDC) consists of tephra ranging in size from boulders to microscopic shards of glass (made up of the edges and junctions of the bubbles of shattered pumice), plus gases (dominated by water vapour, but also including other gases). The temperature of this material can be as high as 1000°C. Among the most famous PDCs are the one that destroyed Pompeii in the year 79 CE, killing an estimated 18,000 people, and the one that destroyed the town of St. Pierre, Martinique, in 1902, killing an estimated 30,000.
The buoyant upper parts of pyroclastic density currents can flow over water, in some cases for several kilometres. The 1902 St. Pierre PDC flowed out into the city's harbour and destroyed several wooden ships anchored there.
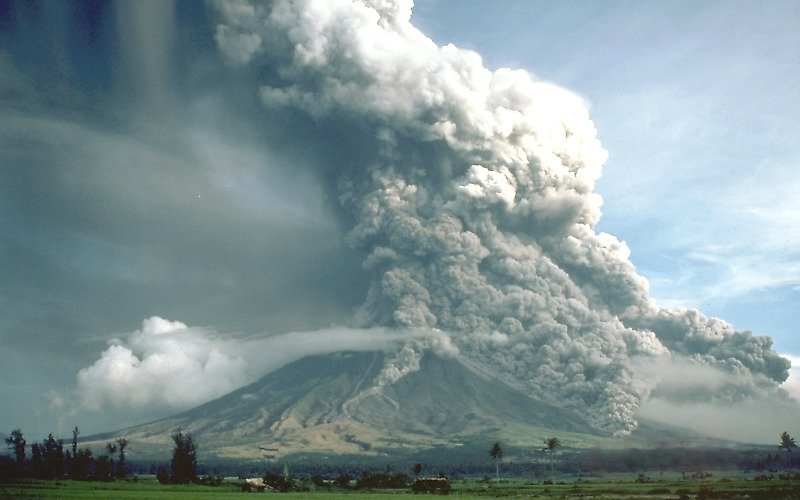
Pyroclastic Fall
Most of the tephra from an explosive eruption ascends high into the atmosphere, and some of it is distributed around Earth by high-altitude winds. The larger components (larger than 0.1 mm) tend to fall relatively close to the volcano, and the amount produced by large eruptions can cause serious damage and casualties. The large 1991 eruption of Mount Pinatubo in the Philippines resulted in the accumulation of tens of centimetres of ash in fields and on rooftops in the surrounding populated region. Heavy typhoon rains that hit the island at the same time added to the weight of the tephra, leading to the collapse of thousands of roofs and to at least 300 of the 700 deaths attributed to the eruption.
Lahar
A lahar is any mudflow or debris flow that is related to a volcano. Most are caused by melting snow and ice during an eruption, as was the case with the lahar that destroyed the Colombian town of Armero in 1985 (described earlier). Lahars can also happen when there is no volcanic eruption, and one of the reasons is that, as we’ve seen, composite volcanoes tend to be weak and easily eroded.
In October 1998, category 5 hurricane Mitch slammed into the coast of central America. Damage was extensive and 19,000 people died, not so much because of high winds but because of intense rainfall—some regions received almost 2 m of rain over a few days! Mudflows and debris flows occurred in many areas, especially in Honduras and Nicaragua. An example is at the Casita Volcano in Nicaragua, where the heavy rains weakened rock and volcanic debris on the upper slopes, resulting in a debris flow that rapidly built in volume as it raced down the steep slope, and then ripped through the towns of El Porvenir and Rolando Rodriguez killing more than 2,000 people (Figure 4.4.2). El Porvenir and Rolando Rodriguez were new towns that had been built without planning approval in an area that was known to be at risk of lahars.
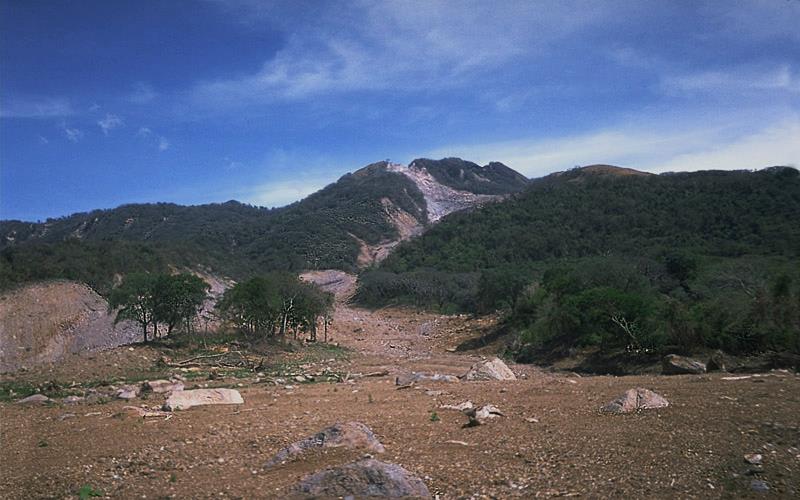
Sector Collapse and Debris Avalanche
In the context of volcanoes, sector collapse or flank collapse is the catastrophic failure of a significant part of an existing volcano, creating a large debris avalanche. This hazard was first recognized with the failure of the north side of Mount St. Helens immediately prior to the large eruption on May 18, 1980. In the weeks before the eruption a large bulge had formed on the side of the volcano, the result of magma transfer from depth into a satellite magma body within the mountain itself. Early on the morning of May 18, a moderate earthquake struck nearby; this is thought to have destabilized the bulge, leading to Earth’s largest ever observed slope failure. The failure of this part of the volcano exposed the underlying satellite magma chamber, causing it to explode sideways, which then exposed the conduit leading to the magma chamber below. The resulting plinian eruption—with a 24 kilometre high eruption column—lasted for nine hours.
In August 2010, a massive part of the flank of B.C.’s Mount Meager gave way and about 48 million cubic metres (m3) of rock rushed down the valley, one of the largest slope failures in Canada in historical times (Figure 4.4.3). More than 25 slope failures have taken place at Mount Meager in the past 8,000 years, some of them more than 10 times larger than the 2010 failure.
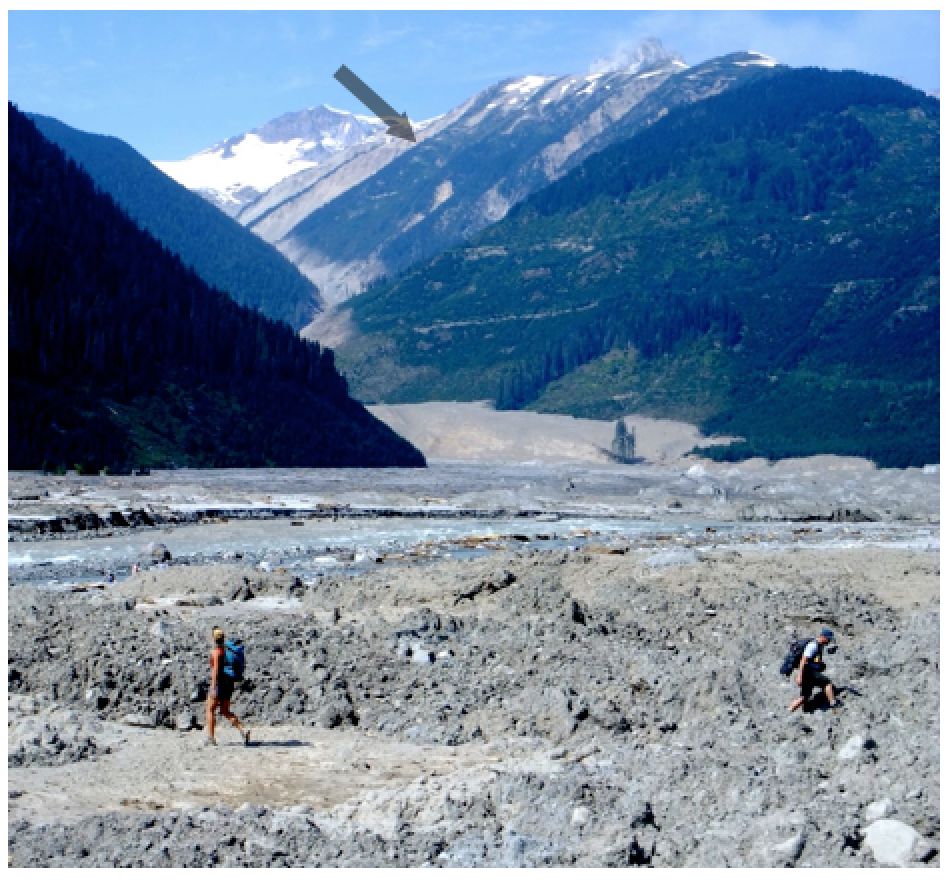
Lava Flows
As we saw in Exercise 4.4, lava flows at volcanoes like Kilauea do not advance very quickly, and in most cases, people can get out of the way. Of course, it is more difficult to move infrastructure, and so buildings and roads are typically the main casualties of lava flows.
Exercise 4.5 Volcanic hazards in Squamish
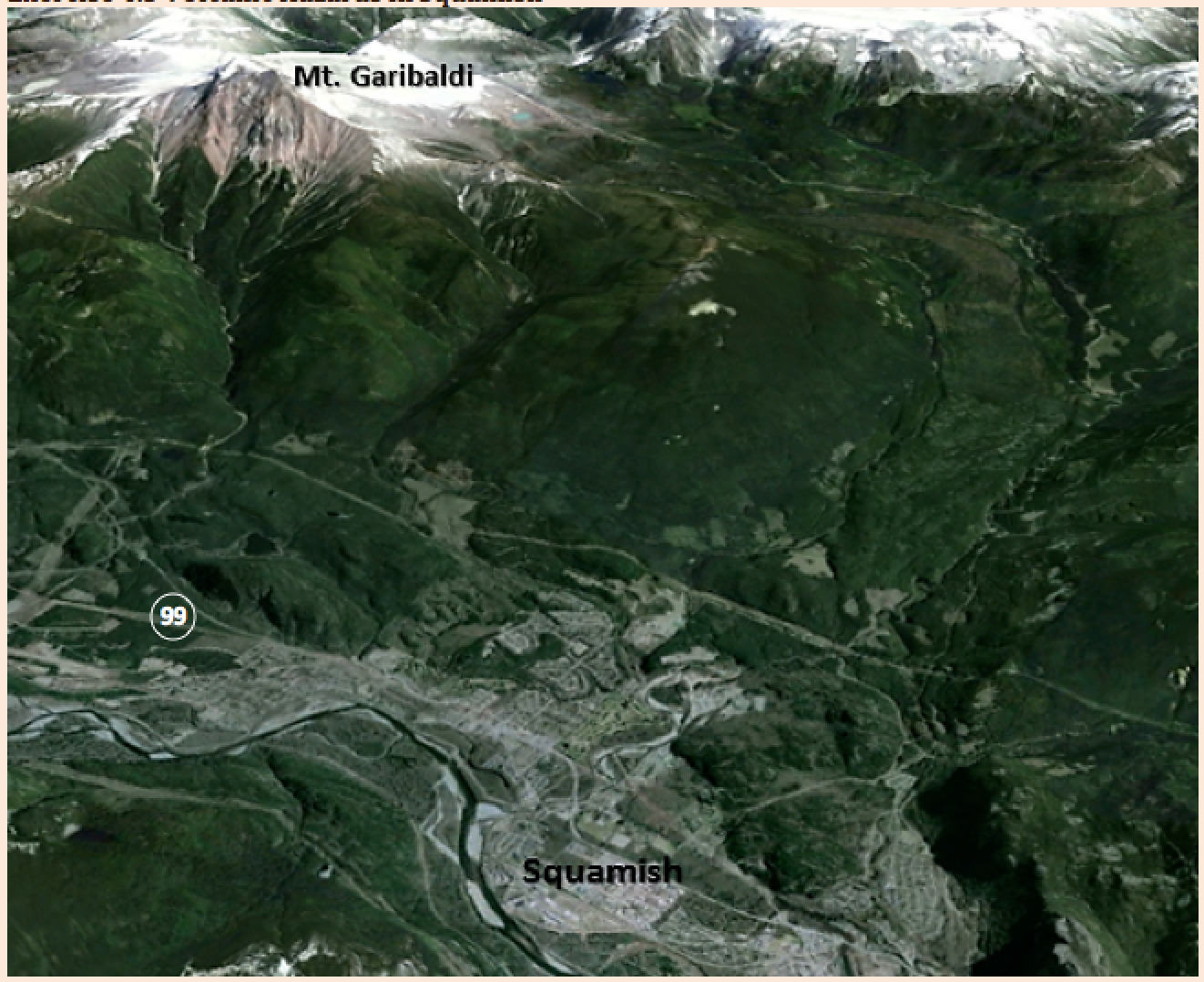
The town of Squamish is situated approximately 10 km from Mount Garibaldi, as shown in the photo. If Mount Garibaldi were to erupt, which of the following hazards could be an issue for people in and around Squamish? Explain why or why not.
- Tephra emission.
- Gas emission.
- Pyroclastic denisty current.
- Pyroclastic fall.
- Lahar.
- Sector collapse.
- Lava flow.
See Appendix 3 for Exercise 4.5 answers.
Media Attributions
- Figure 4.4.1: "Pyroclastic flows at Mayon Volcano" by USGS. Public domain.
- Figure 4.4.2: "Casita Volcano" by USGS. Public domain.
- Figure 4.4.3: August 2010 Mt. Meager landslide, BC © Mika McKinnon. All rights reserved. Used with permission.
- Figure 4.4.4: Screenshot from Google Earth. © Google. Modified by Steve Earle. Used with permission.
In 2005 USGS geologist Chris Newhall made a list of the six most important signs of an imminent volcanic eruption. They are as follows:
- Gas leaks — the release of gases (mostly H2O, CO2, and SO2) from the magma into the atmosphere through cracks in the overlying rock
- Bit of a bulge — the deformation of part of the volcano, indicating that a magma chamber at depth is swelling or becoming more pressurized
- Getting shaky — many (hundreds to thousands) of small earthquakes, indicating that magma is on the move. The quakes may be the result of the magma forcing the surrounding rocks to crack, or a harmonic vibration that is evidence of magmatic fluids moving underground.
- Dropping fast — a sudden decrease in the rate of seismicity, which may indicate that magma has stalled, which could mean that something is about to give way
- Big bump — a pronounced bulge on the side of the volcano (like the one at Mount St. Helens in 1980), which may indicate that magma has moved close to surface
- Blowing off steam — steam eruptions (a.k.a. phreatic eruptions) that happen when magma near the surface heats groundwater to the boiling point. The water eventually explodes, sending fragments of the overlying rock far into the air.
With these signs in mind, we can make a list of the equipment we should have and the actions we can take to monitor a volcano and predict when it might erupt.
Assessing Seismicity
The simplest and cheapest way to monitor a volcano is with seismometers. In an area with several volcanoes that have the potential to erupt (e.g., the Squamish-Pemberton area), a few well-placed seismometers can provide us with an early warning that something is changing beneath one of the volcanoes, and that we need to take a closer look. There are currently enough seismometers in the Lower Mainland and on Vancouver Island to provide this information.[2]
If there is seismic evidence that a volcano is coming to life, more seismometers should be placed in locations within a few tens of kilometres of the source of the activity (Figure 4.5.1). This will allow geologists to determine the exact location and depth of the seismic activity so that they can see where the magma is moving.
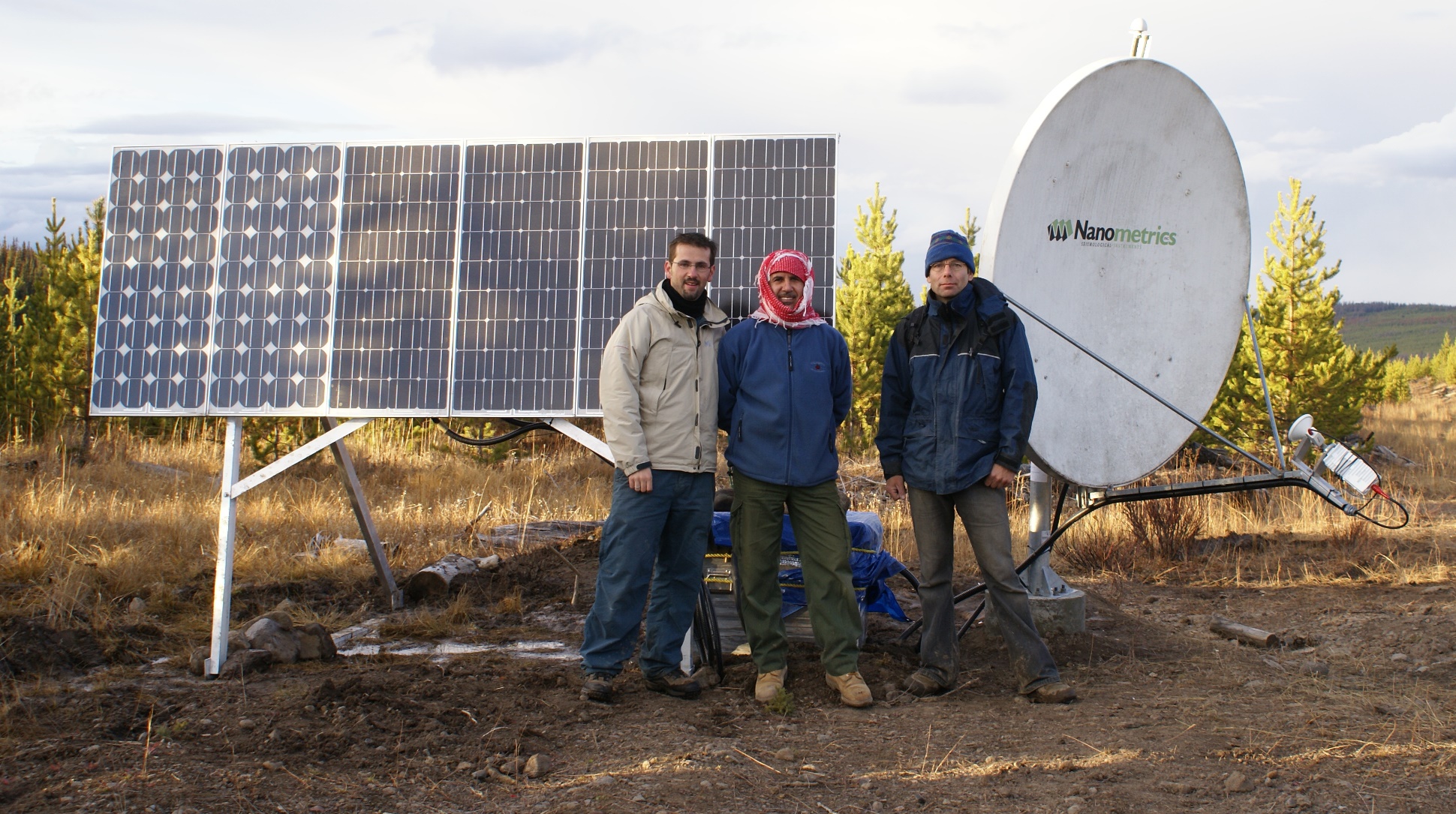
Detecting Gases
Water vapour quickly turns into clouds of liquid water droplets and is relatively easy to detect just by looking, but CO2 and SO2 are not as obvious. It’s important to be able to monitor changes in the composition of volcanic gases, and we need instruments to do that. Some can be monitored from a distance (from the ground or even from the air) using infrared devices, but to obtain more accurate data, we need to sample the air and do chemical analysis. This can be achieved with instruments placed on the ground close to the source of the gases (see Figure 4.3.12), or by collecting samples of the air and analyzing them in a lab.
Measuring Deformation
Measurement of deformation allows us to determine if a volcano is expanding or contracting. That can typically be related to the movement of magma into or out of a magma chamber near to surface and so is an indicator of the potential for an eruption. There are two main ways to measure ground deformation. One is known as a tiltmeter, which is a sensitive three-directional level that can sense small changes in the tilt of the ground at a specific location. Another is through the use of GPS (global positioning system) technology (Figure 4.5.2). Both are effective, but GPS provides more information than a tiltmeter because it can tell us how far the ground has actually moved in the east-west, north-south, and up-down directions.
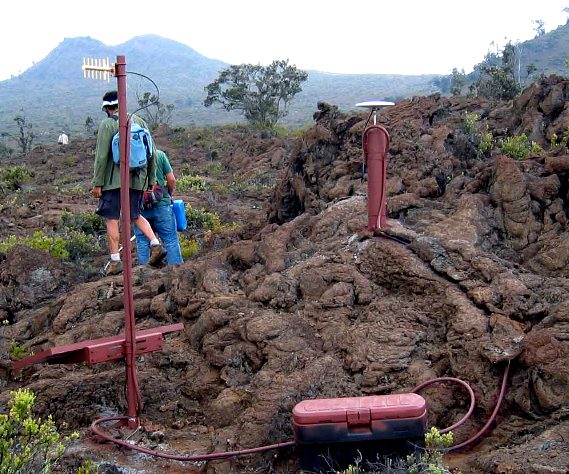
By combining information from these types of sources, along with careful observations made on the ground and from the air, and a thorough knowledge of how volcanoes work, geologists can get a good idea of the potential for a volcano to erupt in the near future (months to weeks, but not days). They can then make recommendations to authorities about the need for evacuations and restricting transportation corridors. Our ability to predict volcanic eruptions has increased dramatically in recent decades because of advances in our understanding of how volcanoes behave and in monitoring technology. Providing that careful work is done, there is no longer a large risk of surprise eruptions, and providing that public warnings are issued and heeded, it is less and less likely that thousands will die from sector collapse, pyroclastic flows, ash falls, or lahars. Indirect hazards are still very real, however, and we can expect the next eruption like the one at Laki in 1783 to take an even greater toll than it did then, especially since there are now roughly eight times as many people on Earth.
Exercise 4.6 Volcano alert!
You’re the chief volcanologist for the Geological Survey of Canada (GSC), based in Vancouver. At 10:30 a.m. on a Tuesday, you receive a report from a seismologist at the GSC in Sidney saying that there has been a sudden increase in the number of small earthquakes in the vicinity of Mount Garibaldi. You have two technicians available, access to some monitoring equipment, and a four-wheel-drive vehicle. At noon, you meet with your technicians and a couple of other geologists. By the end of the day, you need to have a plan to implement, starting tomorrow morning, and a statement to release to the press. What should your first day’s fieldwork include? What should you say later today in your press release?
See Appendix 3 for Exercise 4.6 Answers.
Media Attributions
- Figure 4.5.1: Photo by Cathie Hickson. All rights reserved. Used with permission.
- Figure 4.5.2: A Typical GPS Monitoring Station by USGS. Public domain.